Spatially Variable Effects of Artificially-Created Physical Complexity on Subtidal Benthos
- 1School of Geography, Earth and Environmental Science, University of Plymouth, Plymouth, United Kingdom
- 2APEM Ltd, Stockport, United Kingdom
- 3ECOncrete Tech Ltd, Tel Aviv, Israel
- 4Institute for Marine and Antarctic Science, University of Tasmania, Hobart, TAS, Australia
- 5Sydney Institute of Marine Science, Mosman, NSW, Australia
- 6Department of Biological Sciences, Macquarie University, Sydney, NSW, Australia
- 7Ocean and Earth Science, National Oceanography Centre Southampton, University of Southampton, Southampton, United Kingdom
- 8The Marine Biological Association of the United Kingdom, The Laboratory, Citadel Hill, Plymouth, United Kingdom
- 9School of Biological and Marine Sciences, University of Plymouth, Plymouth, United Kingdom
- 10PML Applications Ltd, Plymouth, United Kingdom
- 11Toulouse School of Management, Toulouse, France
- 12Purpan School of Engineering, University of Toulouse, Toulouse, France
- 13Department of Biological Sciences, National University of Singapore, Singapore, Singapore
In response to the environmental damage caused by urbanization, Nature-based Solutions (NbS) are being implemented to enhance biodiversity and ecosystem processes with mutual benefits for society and nature. Although the field of NbS is flourishing, experiments in different geographic locations and environmental contexts have produced variable results, with knowledge particularly lacking for the subtidal zone. This study tested the effects of physical complexity on colonizing communities in subtidal habitats in two urban locations: (1) Plymouth, United Kingdom (northeast Atlantic) and (2) Tel Aviv, Israel (eastern Mediterranean) for 15- and 12-months, respectively. At each location, physical complexity was manipulated using experimental tiles that were either flat or had 2.5 or 5.0 cm ridges. In Plymouth, biological complexity was also manipulated through seeding tiles with habitat-forming mussels. The effects of the manipulations on taxon and functional richness, and community composition were assessed at both locations, and in Plymouth the survival and size of seeded mussels and abundance and size of recruited mussels were also assessed. Effects of physical complexity differed between locations. Physical complexity did not influence richness or community composition in Plymouth, while in Tel Aviv, there were effects of complexity on community composition. In Plymouth, effects of biological complexity were found with mussel seeding reducing taxon richness, supporting larger recruited mussels, and influencing community composition. Our results suggest that outcomes of NbS experiments are context-dependent and highlight the risk of extrapolating the findings outside of the context in which they were tested.
Introduction
Urbanization and adaptation to climate change are driving irreversible alteration and artificialization of the global coastline (Firth et al., 2016a; Bugnot et al., 2020; Airoldi et al., 2021). The creation of hard artificial structures to support human activities such as shipping and transport, energy extraction and commercial development, as well as to protect valuable human infrastructure from rising seas, has resulted in the modification and loss of natural habitats and associated biodiversity (Halpern et al., 2008; Heery et al., 2017). This is attributed to the footprint of hard artificial structures (e.g., breakwaters, seawalls) which physically replace natural habitat (‘placement loss’; Dugan et al., 2011; Heery et al., 2017). Additionally, in comparison to the natural habitats that they replace, many artificial structures, such as seawalls, have steeper profiles and reduced physical complexity and surface areas, which typically support less diverse biological communities that are often dominated by invasive species (Knott et al., 2004; Moschella et al., 2005; Glasby et al., 2007; Chapman and Underwood, 2011).
In response to global degradation of natural habitats and to support the objectives of the 2030 Agenda for Sustainable Development, The United Nations General Assembly declared 2021–2030 to be the United Nations Decade on Ecosystem Restoration, with the goal to “prevent, halt and reverse the degradation of ecosystems worldwide” (United Nations (UN), 2020). The UN has proposed a wide range of practices, with a particular focus on restoring degraded ecosystems by conserving biodiversity and enhancing ecosystem services. In the context of urban coastal ecosystems, this includes moving beyond traditional hard engineering approaches by working with nature wherever possible (e.g., Hashim et al., 2010; Temmerman et al., 2013; Morris et al., 2019). In heavily urbanized marine settings where space is limited or working with nature is not practical and where hard artificial structures are traditionally used, more sensitive, multi-functional designs that minimize ecological impacts and make space for nature in the built environment should be implemented (Dafforn et al., 2015a; Evans et al., 2017, 2019).
‘Nature-based Solutions’ (NbS) can provide ecologically sustainable options for protecting, restoring and managing natural and modified coastal ecosystems to conserve biodiversity and simultaneously promote human well-being (Eggermont et al., 2015; Raymond et al., 2017; IUCN, 2020). The NbS concept has developed over time as a term to describe many ecologically focused practices such as ecological engineering, restoration and reconciliation (Dick et al., 2019). It ranges from removing built infrastructure to allow for nature to recolonize habitats (‘managed realignment’; French, 2006; Masselink et al., 2017), to enhancing natural features such as sand dunes and oyster reefs (Hanley et al., 2014; Chowdhury et al., 2019) and using integrated green–gray infrastructure (‘IGGI’; Naylor et al., 2017) which incorporates biodiversity and habitat features into both new and existing engineered structures (Firth et al., 2014; Strain et al., 2017a; Morris et al., 2018).
The practice of IGGI can include the provision of habitat complexity (physical or biological), which is a key driver of species diversity (Kostylev et al., 2005; Kovalenko et al., 2012). Complex habitats provide more surface area and a greater diversity of microhabitats (Preston, 1960; Kostylev et al., 2005; but see Loke et al., 2019, who found effects of complexity can be area-independent), both of which can moderate the effects of negative biotic interactions (i.e., competition and predation; Whittaker et al., 1973; Gregor and Anderson, 2016) and abiotic stressors (Connell, 1972; Coombes et al., 2013; Scheffers et al., 2014) underpinning species coexistence (Connell and Slatyer, 1977; Huston and DeAngelis, 1994). The ultimate goal of IGGI is to promote biodiversity and species coexistence which has knock-on benefits for ecosystem functioning (e.g., biofiltration by bivalves; Wilkinson et al., 1996; Vozzo et al., 2021), in turn providing ecosystem services such as regulating (e.g., water purification, Hawkins et al., 2020), supporting (e.g., primary production, Heery et al., 2020) and cultural (Hall et al., 2019) services.
Habitat creation has been used for centuries in subtidal habitats through installation of nearshore and offshore artificial reefs (Baine, 2001; Lima et al., 2019). Artificial reefs add hard substrate, usually of varying substrate complexities, to benthic habitats (typically soft-bottom habitats) for the purposes of enhancing biological communities (Bohnsack and Sutherland, 1985; Baine, 2001; Claisse et al., 2014), which have positive knock-on effects such as water quality improvement (Antsulevich, 1994; Falcão et al., 2009) and provision of recreational activities boosting local tourism (Milon, 1989; Stolk et al., 2007; Herbert et al., 2017). Extensive research has been done on the effectiveness of artificial reef designs to produce biomass and enhance fisheries (Bohnsack and Sutherland, 1985; Claisse et al., 2014; Smith et al., 2016). Research and development of habitat creation in coastal environments, however, is decades behind artificial reef research, but has flourished in recent years (for a review see Strain et al., 2017a). IGGI interventions in coastal environments have ranged from the addition of microtexture (Coombes et al., 2015), crevices and grooves (Martins et al., 2010; Borsje et al., 2011) and artificial rock pools (Evans et al., 2016; Firth et al., 2016b) to existing coastal structures, to the use of precast habitat enhancement units and panels (Browne and Chapman, 2014; Perkol-Finkel et al., 2018; Evans et al., 2021; Strain et al., 2021), all yielding promising biodiversity outcomes. Furthermore, habitat-forming species such as mussels and oysters (Vozzo et al., 2021), corals (Ng et al., 2015) and canopy-forming algae (Falace et al., 2006; Perkol-Finkel et al., 2012), can be seeded onto artificial structures to encourage settlement of conspecifics and increase biodiversity. Still, the majority of IGGI trials have been small-scale, focused on either offshore artificial reefs or coastal intertidal habitats, confined to mostly temperate and occasionally subtropical climates (Strain et al., 2017a), and with few comparisons made among geographic or environmental contexts (but see Hsiung et al., 2020; Strain et al., 2021; Clifton et al., in review). Despite the likely context-dependency of interventions, government agencies and planning authorities are increasingly recommending and implementing integrated green–gray infrastructure strategies as mitigation and compensation for environmental damage caused by new developments (e.g., ‘Biodiversity Net Gain’; Dafforn et al., 2015b; Naylor et al., 2017; Evans et al., 2019). Expanding greening of gray infrastructure techniques to different locations and environmental conditions will improve the evidence base, thus reducing any potential risks of the practice (see Firth et al., 2020a).
Here we analyzed the effects of subtidal habitat complexity on biological colonization of urban artificial structures across two geographic locations: Plymouth, United Kingdom and Tel Aviv, Israel. The overarching aim was to test the effects of physical complexity (using complex tiles with ridges vs. flat tiles with no ridges) on the richness and community composition of colonizing species. Additionally, in Plymouth, we examined how the effect of physical complexity interacts with the effects of biological complexity (manipulated by mussel seeding). We hypothesized that:
(1) Effectiveness of the manipulations would vary between locations;
(2) taxon richness would be higher in treatments with greater physical and/or biological complexity than those without;
(3) community composition would vary with complexity;
(4) and mussel seeding would be more effective in the presence than the absence of physical complexity due to enhanced mussel survival and recruitment (Plymouth only).
Due to the differences in experimental design and environmental conditions between Plymouth and Tel Aviv, formal analyses between the locations were not performed. Differences in experimental design were a result of logistical and contextual site-related constraints, mainly permissions from relevant authorities and space available for experiments, such that the designs had to be sensitive to the main users of the structures. Despite differences in the experiments across the two locations, experiments still had a level of similarity that allowed for the discussion of patterns in the results.
Materials and Methods
Study Sites
Experiments were undertaken in two recreational marinas in Plymouth, United Kingdom (50.364558, –4.172333; 50.359619, –4.120661), which were located approximately 3 km apart, and one marina in Tel Aviv, Israel (32.162333, 34.794083) (Supplementary Figure 1). Plymouth is located in the western English Channel where a large and natural, but heavily urbanized harbor protects predominantly rocky habitats in the outer harbor and sedimentary habitats in the inner estuaries. Despite its long history of anthropogenic alteration, Plymouth Sound supports high marine biodiversity including a number of rare or important habitats and species (Knights et al., 2016). Tel Aviv is located along the most densely populated area on the Israeli Mediterranean coast. The coastline is characterized by a network of marinas and shore-parallel offshore breakwaters located along a predominantly sandy coastline. Arid climate conditions coupled with Tel Aviv’s geographic position in the eastern Mediterranean Sea result in generally low species diversity compared to other parts of the Mediterranean coastline (Azov, 1991). All study sites were located within in sheltered marina conditions.
Experimental Design
Physical complexity (hereafter ‘complexity’) was manipulated using 0.25 m × 0.25 m experimental tiles that were either: (1) flat (without ridges); (2) had 2.5 cm high ridges with associated crevices (hereafter ‘2.5 cm tiles’); or (3) had 5.0 cm high ridges (hereafter ‘5.0 cm tiles’). All tiles had a similar microtexture of <1 mm grooves (for full description of tiles, see Strain et al., 2017b; Supplementary Figure 2).
In Plymouth, ten tiles of each of the three different complexity treatments were deployed at each of the two sites, with five of the ten tiles from each treatment seeded with mussels (Mytilus spp.) (hereafter ‘seeding’). Mussels (potentially a combination of both Mytilus edulis, M. galloprovincialis and hybrids of the two) were used for seeding because they are common habitat-formers on rocky intertidal and shallow subtidal habitats of Plymouth Sound (Hilbish et al., 2002). Tiles assigned to the seeding treatment received 30 mussels (shell length (SL): 32.6 ± 0.1 mm; shell width (SW): 14.4 ± 0.1 mm; mean ± SE), that covered 30–35% of the tile. Mussels were arranged in clusters of three individuals and affixed to tiles using Gorilla® superglue (Cincinnati, OH, United States). On complex tiles, mussels were distributed equally between crevices and ridges. Mussels were collected from rocky lower intertidal habitat in Whitsand Bay (located <10 km west of experimental sites). Tiles were attached to 3.6-m long plastic boards and hung from the south-facing sides of floating pontoons (to maximize light) 1.5 m below the water’s surface facing out from the pontoon (Supplementary Figure 3a). All tiles were attached to boards in the same orientation (ridges running vertically), arranged randomly with respect to treatment, and with 0.4 m spacing between adjacent tiles. Tiles were deployed at both sites in April 2017 and were left in place for 15 months (see Table 1 for experimental design details). Every 3 months during the experiments, water temperature was measured at both sites using a YSI 30 handheld salinity, conductivity and temperature meter (YSI Incorporated, OH, United States).
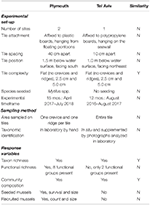
Table 1. Comparison of experimental set-up, sampling methods, and response variables measured in experiments testing effects of physical and biological complexity (mussel seeding) in subtidal habitats in marinas in Plymouth, United Kingdom (NE Atlantic) and Tel Aviv, Israel (eastern Mediterranean).
In Tel Aviv, five tiles of each of the three complexity treatments were deployed, with each tile unseeded. Tiles were deployed randomly with respect to treatment on a polypropylene board with 0.1 m spacing between tiles. The board was hung approximately 1 m below the water surface at a marina seawall facing northeast (Supplementary Figure 3b). Since the mean tidal amplitude in this region is ca. 0.3 m (Einav et al., 1995), the tiles remained fixed at approximately 1 m below the water surface at all times. Experiments in Tel Aviv commenced in August 2016 and were left in place for 12 months (see Table 1 for experimental design details). Every 3 months during the experiment, water temperature was measured using a Sera, D 52518 temperature probe (Sera, Heinsberg, Germany).
Sampling Procedure
In Plymouth, after 15 months (July 2018), all tiles were retrieved, placed in mesh bags (<5 mm holes) to retain all organisms and transported back to the laboratory in cool boxes filled with ambient seawater. Organisms were kept alive until processing (<96 h) by storing tiles in flow-through seawater tanks at ambient conditions, and conditions of the organisms were monitored regularly. Tiles were processed by removing all organisms from one crevice and one ridge on each complex tile, or equivalent areas from flat tiles. The same crevice and ridge were sampled across all tiles. When standardized by area, such subsampling provides estimates of abundance and richness that do not significantly differ from sampling the full tile (Strain et al., 2020). Counts of mobile organisms and percentage cover of sessile organisms were recorded. All organisms were identified to the lowest taxonomic resolution possible, and non-native organisms identified to species level. All seeded and recruited mussels were removed, their position on the tile (i.e., crevice, ridge) was recorded and each individual was measured [shell height and shell width (mm)].
In Tel Aviv, after 12 months (August 2017), tiles were censused in the field following the methods of Perkol-Finkel et al. (2008), which included visual identification and enumeration of mobile organisms and photographing tiles in situ for analysis of percentage cover of sessile organisms using CoralNet software (Beijbom, 2015) in the laboratory. Samples were taken for laboratory identification only when species could not be positively identified in the field. Species were identified to the lowest possible taxonomic level. Tiles were subsampled as described above for the Plymouth experiment.
Statistical Analyses
Due to differences in experimental protocols (see Table 1 for comparison), results between the two locations were not formally statistically compared. Rather, where possible, the results were described in relation to differences in the overall direction of the patterns between locations. Additionally, because experiments in Plymouth incorporated an additional treatment (biological complexity) and were conducted at two sites, statistical analyses were more extensive compared to those done for the experiment in Tel Aviv.
In Plymouth, hypotheses concerning the number and size of seeded (surviving) mussels were tested by running permutational multivariate analysis of variance tests (PERMANOVAs) using square root and fourth root transformed data for number and size of seeded mussels, respectively, after Bray-Curtis similarity matrices were computed. PERMANOVAs included the factors: complexity (fixed; flat, 2.5 cm, 5.0 cm) and site (random; Site 1, 2) and the interaction between these terms.
In Plymouth, taxon and functional richness were quantified using number of taxa and functional groups, respectively. Animals were grouped into functional groups based on their feeding strategies, while algae were grouped following Steneck and Dethier (1994), who grouped marine algae based on morphology and anatomy. Community composition was analyzed using percentage cover of sessile taxa and counts of mobile taxa. All analyses used data collected at the end of the experiment (15 months). Seeded but not recruited mussels were excluded from richness and community composition analyses. To test hypotheses concerning differences in taxon and functional richness and community composition among complexity and seeding in Plymouth, we employed PERMANOVAs using untransformed presence/absence data (richness) on Bray–Curtis similarity matrices, while community composition was analyzed using fourth root transformed abundance data to reduce the influence of very abundant species (Anderson et al., 2008) after Bray–Curtis similarity matrices were computed. Three-way PERMANOVAs were used with factors: complexity (fixed; flat, 2.5 cm, 5.0 cm), seeding (fixed; seeded, unseeded) and site (random; Site 1, 2) and the interaction between these terms. We also investigated the effects of complexity, seeding and site (as above) on the number and size of recruited mussels using PERMANOVAs on log transformed data after Bray–Curtis similarity matrices were computed.
In Tel Aviv, taxon diversity was quantified using taxon richness and community composition data collected at the end of the experiment (12 months) using both in situ counts and photographs. Functional richness was not assessed because there were only two functional groups present on tiles. Differences in taxon richness and community composition among tile treatments were examined using one-way PERMANOVAs with factor complexity (fixed; flat, 2.5 cm, 5.0 cm) after Bray–Curtis similarity matrices were computed. Taxon richness data were left untransformed, while community composition data were square root transformed to reduce the influence of very abundant species (Anderson et al., 2008).
All statistical tests were run in PRIMER v6 with the PERMANOVA + add-on (PRIMER-E Ltd, Plymouth, United Kingdom; Anderson et al., 2008) using the PERMANOVA routine. All transformations were chosen with the aid of the Shade Plot function in PRIMER. For both Plymouth and Tel Aviv, PERMANOVAs were based on 9999 permutations of residuals under a reduced model. Where there were not enough possible permutations to perform a reasonable test (<100 unique permutations), Monte Carlo tests were used to determine significance (Anderson et al., 2008). Post hoc pairwise tests investigated sources of significant treatment effects (at P ≤ 0.05). All multivariate data were visualized using non-metric multi-dimensional scaling (nMDS) plots. Where significant differences in community composition were detected among treatments, percentage contributions of individual taxa to dissimilarities between treatments were analyzed using the similarity percentage (SIMPER) routine (Clarke and Gorley, 2015).
Results
Plymouth: General Overview
Overall, 77 taxa were recorded on tiles, 16 (21%) of which were non-native (Table 2). Fourteen macroalgal taxa, 12 mobile and 51 sessile animals were recorded. The complex (2.5 cm and 5.0 cm) tiles supported similar numbers of taxa (58 and 56, respectively), while flat tiles supported slightly more species (66). Seeded and unseeded tiles supported similar numbers of taxa (65 and 66, respectively). Eight functional groups were present: filter feeders, omnivores, detritivores/scavengers, predators, corticated foliose algae, filamentous algae, leathery macrophytes and corticated macrophytes, with filter feeders the most numerically dominant group (53 taxa). Flat and 5.0 cm complex tiles supported all functional groups. One seeded 5.0 cm tile at Site 1 and one unseeded 5.0 cm tile at Site 2 were lost during the experiment. Water temperature ranged from 5.1 to 24.0°C throughout the experiments, which fell within normal conditions for Plymouth Sound.
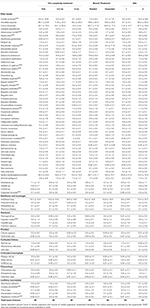
Table 2. Summary table for taxa recorded on subtidal tiles in Plymouth, United Kingdom at the end of 14-month experiments (April 2017–July 2018) testing effects of physical (tile) and biological (mussel) complexity at two sites.
Plymouth: Seeded Mussels
A total of 288 (32%) seeded mussels survived the 15-month experiment, with survival generally greater in crevices (43%) compared to ridges (18%) (Supplementary Table 1). The number and size of surviving mussels did not vary with tile complexity, but size did vary by site (Supplementary Table 2), with Site 2 supporting larger mussels than Site 1.
Plymouth: Richness and Community Composition
Mean taxon richness was unaffected by tile complexity but was significantly greater on unseeded compared to seeded tiles (F1,46 = 58.186, P = 0.0454; Table 3 and Supplementary Table 3a). Mean functional richness did not differ among tile complexity nor seeding treatments (Table 3 and Supplementary Table 3b).
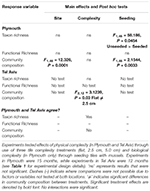
Table 3. Summary of PERMANOVA test results comparing richness (taxon and functional) and community composition from Plymouth, United Kingdom (NE Atlantic) and Tel Aviv, Israel (eastern Mediterranean Sea) and how results compared across locations.
Community composition did not differ among complexity treatments but was significantly different between seeded and unseeded tiles (F1,46 = 2.1544, P = 0.0033; Table 3, Figure 1, and Supplementary Table 4). An analysis of the contribution from individual taxa (SIMPER) showed that approximately 25% of the dissimilarity observed between seeded and unseeded tiles was due to contributions from 7 taxa, all of which were filter feeders. The top contributing taxon was Ascidiella aspersa (4.3%), followed by Styela clava (3.8%), Corella eumyota (3.7%), Cryptosula pallasiana (3.5%), Aplidium cf. glabrum (3.5%), Mytilus spp. (3.4%), and Bugulina fulva (3.2%), with A. aspersa, C. eumyota, and Mytilus spp. each more abundant on seeded tiles, and S. clava, C. pallasiana, and B. fulva each more abundant on unseeded tiles and A. c.f. glabrum in equal abundance. For SIMPER analyses, a good indication that a species consistently contributes to differences between treatments is that the ratio of the average contribution divided by the standard deviation across all pairs of samples (Diss/SD) is >1 (Terlizzi et al., 2005; Clarke and Gorley, 2015). Here we found that, of the taxa that cumulatively contributed to 50% of dissimilarity, all taxa except Bugula neritina, Botrylloides violaceus, Conopeum reticulum, and Ciona intestinalis consistently contributed to differences at Diss/SD > 1, with the most consistent contributor being Spirobranchus sp. (with the taxon more abundant on unseeded tiles) (Supplementary Table 5).
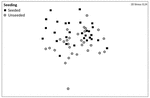
Figure 1. Non-metric multi-dimensional scaling (nMDS) ordination on Bray–Curtis resemblances using fourth root transformed multivariate data showing the effects of mussel seeding (using Mytilus spp.) on community composition at two sites in Plymouth, United Kingdom (NE Atlantic). Community composition data were collected from seeded (denoted by dark squares) and unseeded (gray circles) tiles at the end of the 15-month experiment (April 2017–July 2018).
Plymouth: Recruited Mussels
At the end of the 15-month experiment, 2,702 newly recruited mussels were present on tiles (Supplementary Table 6). The number of recruited mussels did not differ among tile complexity nor seeding treatments (Supplementary Table 7a). A significant three-way interaction (Site × Complexity × Seeding) yielded complex results for mean size of recruited mussels (Supplementary Table 7b), with mean shell lengths significantly higher on seeded compared to unseeded treatments (in four of six comparisons; Figure 2 and Supplementary Table 8), but no clear pattern evident for comparisons among tile complexity treatments. More mussels recruited to Site 1 than Site 2, with larger mussels found on tiles at Site 1 compared to Site 2.
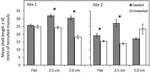
Figure 2. Comparison of mean shell length (mm) ± standard error of mussels that recruited to flat and complex seeded and unseeded tiles at two sites in Plymouth, United Kingdom (NE Atlantic) at the end of 15-month experiments (April 2017–July 2018). Significant interactions among Site x Complexity x Seeding are shown by mussel seeding (seeded, unseeded tiles). Asterisks (∗) indicate significant differences between seeding treatments at P ≤ 0.05.
Tel Aviv: General Overview
At the end of the 12-month experiment, 11 taxa were recorded on experimental tiles, with only one non-native species recorded (the fingerprint oyster, Alectryonella plicatula), which was found on complex tiles only (Supplementary Table 9). 5.0 cm tiles supported 10 of the taxa while 2.5 cm and flat tiles each supported 9. Of these taxa, two were macroalgae and 9 were sessile animals, with no mobile animals recorded (which might be attributed to tiles being sampled in situ). Only two functional groups were recorded at the end of the experiment (filter feeder and primary producer). Water temperature ranged from 15.7 to 30.4°C throughout the experiment, which fell within normal ranges for the eastern Mediterranean Sea.
Tel Aviv: Richness and Community Composition
Mean taxon richness did not vary with tile complexity (Supplementary Table 10a), while community composition did vary significantly with complexity (F2,12 = 3.1239, P = 0.0300; Figure 3 and Supplementary Table 10b), with post hoc pairwise comparisons revealing significant differences between flat and 2.5 cm tiles. Seventy-five percent of the dissimilarities between these tiles were attributed to only four taxa. Turf algae contributed the most (46.0%), followed by Spirorbis spp. (11.1%), Ostrea edulis (10.0%), and an unidentified encrusting bryozoan (9.8%), with all taxa more abundant on flat tiles except for O. edulis. All four taxa showed consistently high contribution to dissimilarities between treatments (Diss/SD > 1), with O. edulis being the most consistent contributor (Supplementary Table 11).
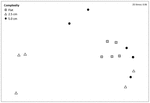
Figure 3. Non-metric multi-dimensional scaling (nMDS) ordination on Bray–Curtis resemblances using square root transformed multivariate data showing the effects of physical complexity on community composition at a marina in Tel Aviv, Israel (eastern Mediterranean Sea). Community data were collected from subtidal flat tiles (without crevice and ridges), 2.5 cm complexity tiles and 5.0 cm complexity tiles at the end of the 12-month experiment (August 2016–August 2017).
Discussion
In contrast to our hypotheses, this study showed little evidence of biodiversity benefits as a result of increasing either physical or biological complexity in subtidal habitats. Despite finding no significant differences in mean taxon richness across tile complexities at either location, in Plymouth, the physically complex tiles with ridges actually supported lower total numbers of taxa (56 and 58) than the flat tiles with no ridges (66 taxa), with four of the 16 non-native taxa found only on flat tiles (Table 2). Furthermore, the biologically complex tiles that were seeded with mussels supported significantly lower mean taxon richness than tiles that were unseeded. The only finding that agreed with our hypotheses was that recruited mussels were bigger on the biologically complex tiles that were seeded compared to those that recruited to unseeded tiles.
This study replicated methods used by previous intertidal experiments in locations across the globe, including Plymouth and Tel Aviv (Strain et al., 2021). In Plymouth, we found similar effects of physical complexity in the subtidal zone as previously found in the intertidal (Strain et al., 2021), with physical complexity having no detectable influence on taxon richness in either instance. In Tel Aviv, however, intertidal trials revealed positive effects of physical complexity (Strain et al., 2021) that contrasted the neutral effects found in the subtidal here. Physical complexity in the intertidal zone was likely more important for reducing thermal stress in Tel Aviv, which is characterized by dry and hot conditions (approximately 500 mm mean annual rainfall; 14–26°C air temperature; Striem, 1967; Azov, 1991) compared to Plymouth, which is characterized by rainy conditions and a temperate climate (approximately 1000 mm mean annual rainfall; 6–16°C air temperature; Barrow and Hulme, 1997; Murphy et al., 2019). The differing effects of physical complexity between our subtidal experiments and previous intertidal experiments likely arose from differences in the key environmental stressors to which subtidal and intertidal communities are exposed.
In subtidal habitats, research on artificial reefs generally shows increased diversity with the addition of habitat (Bohnsack and Sutherland, 1985), which is counter to results from the current study. Although also located in the subtidal, it is challenging to directly compare artificial reefs to coastal subtidal habitats, as artificial reefs are positioned in nearshore or deeper offshore waters which experience different environmental conditions compared to coastal subtidal habitats. Furthermore, artificial reef studies typically focus on production versus attraction of reef and game fish as well as fisheries potential of the reef (Pickering and Whitmarsh, 1997; Watanuki and Gonzales, 2006), with few studies analyzing the fouling communities (Baine, 2001). Despite these differences, ecologists developing subtidal habitat enhancements in coastal environments can still be able to learn from the decades of research on nearshore and offshore artificial reefs (Firth et al., 2020a).
Positive effects of physical and biological complexity on intertidal biodiversity (e.g., Vozzo et al., 2021) have, in part, been attributed to the amelioration of low tide air temperature extremes and desiccation stress by complex microhabitats (see McAfee et al., 2016, 2017) as well as the protection these provide from predators (Martins et al., 2010; Strain et al., 2017b). In subtidal environments, small-scale physical and biological complexity, however, have little influence on water temperature or on physico-chemical stressors related to water quality. Instead, the provision of biological complexity may competitively exclude species through pre-emption of space (Underwood, 2000; Bateman and Bishop, 2017), reducing food availability (e.g., mussels reducing food resources for other filter feeders; Peterson and Beal, 1989) or negatively influencing settlement of organisms through passive ingestion of larvae through filter feeding (Lehane and Davenport, 2004; Porri et al., 2008). In Plymouth, there was a negative effect of biological complexity on taxon richness and no effect of biological complexity on the numbers of recruited mussels, contrary to the generally positive effects of bivalves on biodiversity and recruitment of conspecifics in the intertidal zone (Bradford et al., 2020; Vozzo et al., 2021). As the current study only assessed mussel recruitment after 15 months, it is possible that mussel seeding initially had a positive effect on mussel recruitment, but subsequent density dependent mortality had counteracted this treatment effect by 15 months (Benedetti-Cecchi, 2000; Maggi et al., 2011).
Whether complexity in subtidal habitats provides protection from predation (e.g., Connell and Anderson, 1999; Ferrario et al., 2016) will be dependent on the match between the dimensions of the microhabitats provided and the body size of key predators. The body sizes of predators can determine access to prey within microhabitats (Hacker and Steneck, 1990; Strain et al., 2017b). As physical complexity had no effect on taxon richness in either Plymouth or Tel Aviv, it is likely that colonizing organisms on both flat and complex tiles were equally affected by predators. This may be explained by low rates of predation that exert little top–down control on community structure, or alternatively, a small body size of key predators, which allowed them to access not only flat surfaces but also protective crevices. For example, predators of biota on tiles in Plymouth are likely common inshore fish (e.g., mackerel, pollack, mullet), which are small enough to access the crevices of the tiles; while in Tel Aviv, predators are probably crab species and small fish such as croaker, which have feeding strategies that allow them access to the small crevices of the tiles.
Of the species contributing most to observed differences in community composition between seeded and unseeded tiles in Plymouth, 35% were non-native, with over 50% equally or more abundant on seeded tiles. This contrasts with Vozzo et al. (2021) who showed that the proportion of non-native to native species on tiles seeded with oysters was generally lower compared to unseeded tiles in intertidal experiments. As artificial substrata that is constantly submerged throughout the tidal cycle (i.e., subtidal and lower intertidal habitats) has been shown to support greater numbers of non-native species compared to intertidal substrata (O’Shaughnessy, 2020) and natural rocky reefs (Dafforn et al., 2012; Airoldi et al., 2015), there are concerns that addition of substrate (either physical or biological) in the name of IGGI in subtidal habitats may facilitate non-native species spread (Dafforn et al., 2012; Evans et al., 2017; O’Shaughnessy et al., 2020). Additionally, there is growing evidence that invasive species are more likely to settle preferentially on habitat-formers than on the surrounding substrate (Reise, 1998; Firth et al., 2020b). With increasing interest in the use of habitat-forming species for restoration and rehabilitation efforts (Byers et al., 2006; Perkol-Finkel et al., 2012; Ramus et al., 2017), a greater understanding of the role of habitat-forming species in providing novel space for attachment of non-native species in the subtidal is required (Sotka and Byers, 2019).
Although methodological and temporal differences could have contributed to the differing effects of physical complexity between our study locations, our results nevertheless support growing assertions that the effects of complexity are context-dependent (Strain et al., 2021; Clifton et al., in review). The key to maximizing ecological benefits through greening gray infrastructure is to understand specific stressors that influence the colonizing community, and hence the interventions that will most effectively mitigate these. As such, the practice of IGGI would benefit from future experiments investigating how biotic factors such as propagule and larval supply, competition and predator-prey interactions, as well as abiotic factors such as pollution load and wave action interact with complexity. Experiments with standardized methodologies and sampling, longer trials that last years rather than months and regular monitoring of effects are needed to enhance our understanding of ecological responses to IGGI practices over time. It is also important to understand how habitat enhancements affect biodiversity compared to adjacent natural habitat (i.e., spill over onto natural rocky shores) as well as artificial structures with no enhancements (e.g., seawalls).
Conclusion
The success of any habitat enhancement intervention will ultimately depend on well-informed planning underpinned by sound evidence, as well as setting and monitoring of secondary management goals. As such, it is vital to understand which factors influence specific response variables and under which environmental conditions interventions will have the greatest positive effects so that interventions can maximize ecological outcomes and provide an effective tool for achieving management goals (Dafforn et al., 2015b; Mayer-Pinto et al., 2019). Here we show that the drivers of colonization were different between geographic locations, with physical complexity influencing the colonizing community in Tel Aviv but not in Plymouth. The additional test using mussel seeding in Plymouth allowed us to show that biological complexity influenced differences in colonization at this location. We also demonstrate that results from habitat enhancement approaches that have proven positive ecological effects in the intertidal zone do not necessarily translate to subtidal habitats, indicating that integrated green–gray infrastructure is not a ‘one-size-fits-all’ approach. Artificial structures will never be exact surrogates for the natural habitats they replace, and even the best integrated green–gray infrastructure designs cannot replicate nature. However, if nature-based approaches are underpinned by proof-of-concept evidence within the same physical and environmental context in which they are implemented, interventions may have the potential to support greater biodiversity and provide valuable ecosystem services; a true ‘win–win’ for both humans and nature.
Dedication
This work is dedicated to the memory of Shimrit Perkol-Finkel, who was an inspirational pioneer in the field of sustainable coastal engineering. She will be sorely missed.
Data Availability Statement
The original contributions presented in the study are included in the article/Supplementary Material, further inquiries can be directed to the corresponding authors.
Author Contributions
KO’S: methodology, data collection, data analysis, and writing of all drafts. SP-F, TH, and RS: methodology, data collection, and review and editing of drafts. ES and MB: conceptualization, methodology, investigation, funding acquisition, and review and editing of drafts. SH, MH, PL, and RT: methodology, review and editing of drafts, and supervision. AY: data collection, validation, and review and editing of drafts. AA, LM, and CY: data collection and review and editing of drafts. LF: conceptualization, methodology, investigation, review and editing of drafts, funding acquisition, data analysis, and supervision. All authors contributed to the article and approved the submitted version.
Funding
This study was part of the World Harbour Project. ES and MB were supported by funding from The Ian Potter Foundation, Harding Miller Foundation, and the New South Wales Government Office of Science and Research. KO’S was supported by funding from the University of Plymouth.
Conflict of Interest
SP-F, TH, and RS were employed by the company ECOncrete Tech Ltd. AY was employed by the company PML Applications Ltd. KO’S is currently employed by the company APEM Ltd. but did not work for this company at the time of conducting this research.
The remaining authors declare that the research was conducted in the absence of any commercial or financial relationships that could be construed as a potential conflict of interest.
Publisher’s Note
All claims expressed in this article are solely those of the authors and do not necessarily represent those of their affiliated organizations, or those of the publisher, the editors and the reviewers. Any product that may be evaluated in this article, or claim that may be made by its manufacturer, is not guaranteed or endorsed by the publisher.
Acknowledgments
We are tremendously grateful to R. Ticehurst, R. Haslam, R. Kenyon, R. Lilley, J. Ducker, M. Rapsey, and A. Heathman for help with fieldwork. Thanks also to A. James and the University of Plymouth Marine Station for access to laboratory facilities.
Supplementary Material
The Supplementary Material for this article can be found online at: https://www.frontiersin.org/articles/10.3389/fevo.2021.690413/full#supplementary-material
References
Airoldi, L., Beck, M. W., Firth, L. B., Bugnot, A. B., Steinberg, P. D., and Dafforn, K. A. (2021). Emerging solutions to return nature to the urban ocean. Annu. Rev. Mar. Sci. 13, 445–477. doi: 10.1146/annurev-marine-032020-020015
Airoldi, L., Turon, X., Perkol-Finkel, S., and Rius, M. (2015). Corridors for aliens but not for natives: effects of marine urban sprawl at a regional scale. Divers. Distrib. 21, 755–768. doi: 10.1111/ddi.12301
Anderson, M., Gorley, R. N., and Clarke, R. K. (2008). Permanova+ for Primer: guide to Software and Statistical Methods. Plymouth: Primer-E Limited.
Antsulevich, A. E. (1994). Artificial reefs project for improvement of water quality and environmental enhancement of Neva Bay (St.-Petersburg County Region). Bull. Mar. Sci. 55, 1189–1192.
Azov, Y. (1991). Eastern Mediterranean—a marine desert? Mar. Pollut. Bull. 23, 225–232. doi: 10.1016/0025-326X(91)90679-M
Baine, M. (2001). Artificial reefs: a review of their design, application, management and performance. Ocean Coast. Manag. 44, 241–259. doi: 10.1016/S0964-5691(01)00048-5
Barrow, E., and Hulme, M. (1997). Describing the Surface Climate of the British Isles. UK: Routledge.
Bateman, D. C., and Bishop, M. J. (2017). The environmental context and traits of habitat-forming bivalves influence the magnitude of their ecosystem engineering. Mar. Ecol. Progr. Ser. 563, 95–110. doi: 10.3354/meps11959
Benedetti-Cecchi, L. (2000). Predicting direct and indirect interactions during succession in a mid-littoral rocky shore assemblage. Ecol. Monogr. 70, 45–72.
Bohnsack, J. A., and Sutherland, D. L. (1985). Artificial reef research: a review with recommendations for future priorities. Bull. Mar. Sci. 37, 11–39.
Borsje, B. W., van Wesenbeeck, B. K., Dekker, F., Paalvast, P., Bouma, T. J., van Katwijk, M. M., et al. (2011). How ecological engineering can serve in coastal protection. Ecol. Eng. 37, 113–122. doi: 10.1016/j.ecoleng.2010.11.027
Bradford, T. E., Astudillo, J. C., Lau, E. T., Perkins, M. J., Lo, C. C., and Li, T. C. (2020). Provision of refugia and seeding with native bivalves can enhance biodiversity on vertical seawalls. Mar. Pollut. Bull. 160:111578. doi: 10.1016/j.marpolbul.2020.111578
Browne, M. A., and Chapman, M. G. (2014). Mitigating against the loss of species by adding artificial intertidal pools to existing seawalls. Mar. Ecol. Progr. Ser. 497, 119–129. doi: 10.3354/meps10596
Bugnot, A. B., Mayer-Pinto, M., Airoldi, L., Heery, E. C., Johnston, E. L., Critchley, L. P., et al. (2020). Current and projected global extent of marine built structures. Nat. Sustain. 4, 33–41. doi: 10.1038/s41893-020-00595-1
Byers, J. E., Cuddington, K., Jones, C. G., Talley, T. S., Hastings, A., Lambrinos, J. G., et al. (2006). Using ecosystem engineers to restore ecological systems. Trends Ecol. Evol. 21, 493–500. doi: 10.1016/j.tree.2006.06.002
Chapman, M. G., and Underwood, A. J. (2011). Evaluation of ecological engineering of “armoured” shorelines to improve their value as habitat. J. Exp. Mar. Biol. Ecol. 400, 302–313. doi: 10.1016/j.jembe.2011.02.025
Chowdhury, M. S. N., Walles, B., Sharifuzzaman, S. M., Hossain, M. S., Ysebaert, T., and Smaal, A. C. (2019). Oyster breakwater reefs promote adjacent mudflat stability and salt marsh growth in a monsoon dominated subtropical coast. Sci. Rep. 9, 1–12. doi: 10.1038/s41598-019-44925-6
Claisse, J. T., Pondella, D. J., Love, M., Zahn, L. A., Williams, C. M., Williams, J. P., et al. (2014). Oil platforms off California are among the most productive marine fish habitats globally. Proc. Natl. Acad. Sci. 111, 15462–15467. doi: 10.1073/pnas.1411477111
Clifton, G., Dafforn, K. A., and Bishop, M. J. (in review). The ecological benefits of adding complexity to seawalls vary across estuarine gradients. Ecol. Eng.
Connell, J. H. (1972). Community interactions on marine rocky intertidal shores. Annu. Rev. Ecol. Syst. 3, 169–192.
Connell, J. H., and Slatyer, R. O. (1977). Mechanisms of succession in natural communities and their role in community stability and organization. Am. Nat. 111, 1119–1144. doi: 10.1086/283241
Connell, S. D., and Anderson, M. J. (1999). Predation by fish on assemblages of intertidal epibiota: effects of predator size and patch size. J. Exp. Mar. Biol. Ecol. 241, 15–29. doi: 10.1016/S0022-0981(99)00067-2
Coombes, M. A., La Marca, E. C., Naylor, L. A., and Thompson, R. C. (2015). Getting into the groove: opportunities to enhance the ecological value of hard coastal infrastructure using fine-scale surface textures. Ecol. Eng. 77, 314–323. doi: 10.1016/j.ecoleng.2015.01.032
Coombes, M. A., Naylor, L. A., Viles, H. A., and Thompson, R. C. (2013). Bioprotection and disturbance: seaweed, microclimatic stability and conditions for mechanical weathering in the intertidal zone. Geomorphology 202, 4–14. doi: 10.1016/j.geomorph.2012.09.014
Dafforn, K. A., Glasby, T. M., Airoldi, L., Rivero, N. K., Mayer-Pinto, M., and Johnston, E. L. (2015a). Marine urbanization: an ecological framework for designing multifunctional artificial structures. Front. Ecol. Environ. 13:150108070013002. doi: 10.1890/140050
Dafforn, K. A., Mayer-Pinto, M., Morris, R. L., and Waltham, N. J. (2015b). Application of management tools to integrate ecological principles with the design of marine infrastructure. J. Environ. Manag. 158, 61–73. doi: 10.1016/j.jenvman.2015.05.001
Dafforn, K. A., Glasby, T. M., and Johnston, E. L. (2012). Comparing the invasibility of experimental reefs with field observations of natural reefs and artificial structures. PLoS One 7:e38124. doi: 10.1371/journal.pone.0038124
Dick, J., Miller, J. D., Carruthers-Jones, J., Dobel, A. J., Carver, S., Garbutt, A., et al. (2019). How are nature-based solutions contributing to priority societal challenges surrounding human well-being in the United Kingdom: a systematic map protocol. Environ. Evid. 8:37. doi: 10.1186/s13750-019-0180-4
Dugan, J., Airoldi, L., Chapman, M., Walker, S., and Schlacher, T. (2011). Estuarine and coastal structures: environmental effects, a focus on shore and nearshore structures. Treat. Estuar. Coast. Sci. 8, 17–41. doi: 10.1016/B978-0-12-374711-2.00802-0
Eggermont, H., Balian, E., Azevedo, J. M. N., Beumer, V., Brodin, T., Claudet, J., et al. (2015). Nature-based solutions: new influence for environmental management and research in Europe. GAIA 24, 243–248. doi: 10.14512/gaia.24.4.9
Einav, R., Breckle, S., and Beer, S. (1995). Ecophysiological adaptation strategies of some intertidal marine macroalgae of the Israeli Mediterranean coast. Mar. Ecol. Prog. Ser. 125, 219–228. doi: 10.3354/meps125219
Evans, A. J., Firth, L. B., Hawkins, S. J., Hall, A. E., Ironside, J. E., Thompson, R. C., et al. (2019). From ocean sprawl to blue-green infrastructure–A UK perspective on an issue of global significance. Environ. Sci. Policy 91, 60–69. doi: 10.1016/j.envsci.2018.09.008
Evans, A. J., Firth, L. B., Hawkins, S. J., Morris, E. S., Goudge, H., and Moore, P. J. (2016). Drill-cored rock pools: an effective method of ecological enhancement on artificial structures. Mar. Freshw. Res. 67, 123–130. doi: 10.1071/MF14244
Evans, A. J., Garrod, B., Firth, L. B., Hawkins, S. J., Morris-Webb, E. S., Goudge, H., et al. (2017). Stakeholder priorities for multi-functional coastal defence developments and steps to effective implementation. Mar. Policy 75, 143–155. doi: 10.1016/j.marpol.2016.10.006
Evans, A. J., Lawrence, P. J., Natanzi, A. S., Moore, P. J., Davies, A. J., and Crowe, T. P. (2021). Replicating natural topography on marine artificial structures–A novel approach to eco-engineering. Ecol. Eng. 160:106144. doi: 10.1016/j.ecoleng.2020.106144
Falace, A., Zanelli, E., and Bressan, G. (2006). Algal transplantation as a potential tool for artificial reef management and environmental mitigation. Bull. Mar. Sci. 78, 161–166.
Falcão, M., Santos, M. N., Drago, T., Serpa, D., and Monteiro, C. (2009). Effect of artificial reefs (southern Portugal) on sediment–water transport of nutrients: importance of the hydrodynamic regime. Estuar. Coast. Shelf Sci. 83, 451–459. doi: 10.1016/j.ecss.2009.04.028
Ferrario, F., Iveša, L., Jaklin, A., Perkol-Finkel, S., and Airoldi, L. (2016). The overlooked role of biotic factors in controlling the ecological performance of artificial marine habitats. J. Appl. Ecol. 53, 16–24. doi: 10.1111/1365-2664.12533
Firth, L. B., Airoldi, L., Bulleri, F., Challinor, S., Chee, S. Y., Evans, A. J., et al. (2020a). Greening of grey infrastructure should not be used as a Trojan horse to facilitate coastal development. J. Appl. Ecol. 57, 1762–1768. doi: 10.1111/1365-2664.13683
Firth, L. B., Duff, L., Gribben, P. E., and Knights, A. M. (2020b). Do positive interactions between marine invaders increase likelihood of invasion into natural and artificial habitats? Oikos 130, 453–463. doi: 10.1111/oik.07862
Firth, L. B., Knights, A. M., Bridger, D., Evans, A. J., Mieszkowska, N., Hawkins, S. J., et al. (2016a). Ocean sprawl: challenges and opportunities for biodiversity management in a changing world. Oceanogr. Mar. Biol. Annu. Rev. 54, 189–262. doi: 10.1201/9781315368597
Firth, L. B., Browne, K. A., Knights, A. M., Hawkins, S. J., and Nash, R. (2016b). Eco-engineered rock pools: a concrete solution to biodiversity loss and urban sprawl in the marine environment. Environ. Res. Lett. 11:094015.
Firth, L. B., Thompson, R. C., Bohn, K., Abbiati, M., Airoldi, L., Bouma, T. J., et al. (2014). Between a rock and a hard place: environmental and engineering considerations when designing coastal defence structures. Coast. Eng. 87, 122–135. doi: 10.1016/j.coastaleng.2013.10.015
French, P. W. (2006). Managed realignment–the developing story of a comparatively new approach to soft engineering. Estuar. Coast. Shelf Sci. 67, 409–423. doi: 10.1016/j.ecss.2005.11.035
Glasby, T. M., Connell, S. D., Holloway, M. G., and Hewitt, C. L. (2007). Nonindigenous biota on artificial structures: could habitat creation facilitate biological invasions? Mar. Biol. 151, 887–895. doi: 10.1007/s00227-006-0552-5
Gregor, C. A., and Anderson, T. W. (2016). Relative importance of habitat attributes to predation risk in a temperate reef fish. Environ. Biol. Fishe. 99, 539–556. doi: 10.1007/s10641-016-0496-7
Hacker, S. D., and Steneck, R. S. (1990). Habitat architecture and the abundance and body-size-dependent habitat selection of a phytal amphipod. Ecology 71, 2269–2285. doi: 10.2307/1938638
Hall, A. E., Herbert, R. J., Britton, J. R., Boyd, I. M., and George, N. C. (2019). Shelving the coast with vertipools: retrofitting artificial rock pools on coastal structures as mitigation for coastal squeeze. Front. Mar. Sci. 6:456. doi: 10.3389/fmars.2019.00456
Halpern, B. S., Walbridge, S., Selkoe, K. A., Kappel, C. V., Micheli, F., D’agrosa, C., et al. (2008). A global map of human impact on marine ecosystems. Science 319, 948–952. doi: 10.1126/science.1149345
Hanley, M. E., Hoggart, S. P. G., Simmonds, D. J., Bichot, A., Colangelo, M. A., Bozzeda, F., et al. (2014). Shifting sands? Coastal protection by sand banks, beaches and dunes. Coast. Eng. 87, 136–146. doi: 10.1016/j.coastaleng.2013.10.020
Hashim, R., Kamali, B., Tamin, N. M., and Zakaria, R. (2010). An integrated approach to coastal rehabilitation: mangrove restoration in Sungai Haji Dorani. Malaysia. Estuar. Coast. Shelf Sci. 86, 118–124. doi: 10.1016/j.ecss.2009.10.021
Hawkins, S. J., O’Shaughnessy, K. A., Adams, L. A., Langston, W. J., Bray, S., Allen, J. R., et al. (2020). Recovery of an urbanised estuary: clean-up, de-industrialisation and restoration of redundant dock-basins in the Mersey. Mar. Pollut. Bull. 156:111150. doi: 10.1016/j.marpolbul.2020.111150
Heery, E. C., Bishop, M. J., Critchley, L. P., Bugnot, A. B., Airoldi, L., Mayer-Pinto, M., et al. (2017). Identifying the consequences of ocean sprawl for sedimentary habitats. J. Exp. Mar. Biol. Ecol. 492, 31–48. doi: 10.1016/j.jembe.2017.01.020
Heery, E. C., Lian, K. Y., Loke, L. H., Tan, H. T., and Todd, P. A. (2020). Evaluating seaweed farming as an eco-engineering strategy for ‘blue’shoreline infrastructure. Ecol. Eng. 152:105857. doi: 10.1016/j.ecoleng.2020.105857
Herbert, R. J., Collins, K., Mallinson, J., Hall, A. E., Pegg, J., Ross, K., et al. (2017). Epibenthic and mobile species colonisation of a geotextile artificial surf reef on the south coast of England. PLoS One 12:e0184100. doi: 10.1371/journal.pone.0184100
Hilbish, T., Carson, E., Plante, J., Weaver, L., and Gilg, M. (2002). Distribution of Mytilus edulis, M. galloprovincialis, and their hybrids in open-coast populations of mussels in southwestern England. Mar. Biol. 140, 137–142. doi: 10.1007/s002270100631
Hsiung, A. R., Tan, W. T., Loke, L. H., Firth, L. B., Heery, E. C., Ducker, J., et al. (2020). Little evidence that lowering the pH of concrete supports greater biodiversity on tropical and temperate seawalls. Mar. Ecol. Prog. Ser. 656, 193–205. doi: 10.3354/meps13365
Huston, M. A., and DeAngelis, D. L. (1994). Competition and coexistence: the effects of resource transport and supply rates. Am. Nat. 144, 954–977.
IUCN. (2020). International Union for Conservation of Nature Global Standard for Nature-based Solutions. Switzerland: IUCN.
Knights, A. M., Firth, L. B., Thompson, R. C., Yunnie, A. L., Hiscock, K., and Hawkins, S. J. (2016). Plymouth—A world harbour through the ages. Reg. Stud. Mar. Sci. 8, 297–307. doi: 10.1016/j.rsma.2016.02.002
Knott, N. A., Underwood, A. J., Chapman, M. G., and Glasby, T. M. (2004). Epibiota on vertical and on horizontal surfaces on natural reefs and on artificial structures. J. Mar. Biol. Assoc. U. K. 84, 1117–1130. doi: 10.1017/S0025315404010550h
Kostylev, V. E., Erlandsson, J., Ming, M. Y., and Williams, G. A. (2005). The relative importance of habitat complexity and surface area in assessing biodiversity: fractal application on rocky shores. Ecol. Complex. 2, 272–286. doi: 10.1016/j.ecocom.2005.04.002
Kovalenko, K. E., Thomaz, S. M., and Warfe, D. M. (2012). Habitat complexity: approaches and future directions. Hydrobiologia 685, 1–17. doi: 10.1007/s10750-011-0974-z
Lehane, C., and Davenport, J. (2004). Ingestion of bivalve larvae by Mytilus edulis: experimental and field demonstrations of larviphagy in farmed blue mussels. Mar. Biol. 145, 101–107. doi: 10.1007/s00227-003-1290-6
Lima, J. S., Zalmon, I. R., and Love, M. (2019). Overview and trends of ecological and socioeconomic research on artificial reefs. Mar. Environ. Res. 145, 81–96. doi: 10.1016/j.marenvres.2019.01.010
Loke, L. H., Heery, E. C., Lai, S., Bouma, T. J., and Todd, P. A. (2019). Area-independent effects of water-retaining features on intertidal biodiversity on eco-engineered seawalls in the tropics. Front. Mar. Sci. 6:16. doi: 10.3389/fmars.2019.00016
Maggi, E., Bertocci, I., Vaselli, S., and Benedetti-Cecchi, L. (2011). Connell and Slatyer’s models of succession in the biodiversity era. Ecology 92, 1399–1406. doi: 10.1890/10-1323.1
Martins, G. M., Thompson, R. C., Neto, A. I., Hawkins, S. J., and Jenkins, S. R. (2010). Enhancing stocks of the exploited limpet Patella candei d’Orbigny via modifications in coastal engineering. Biol. Conserv. 143, 203–211. doi: 10.1016/j.biocon.2009.10.004
Masselink, G., Hanley, M. E., Halwyn, A. C., Blake, W., Kingston, K., Newton, T., et al. (2017). Evaluation of salt marsh restoration by means of self-regulating tidal gate - Avon estuary, South Devon, UK. Ecol. Eng. 106, 174–190. doi: 10.1016/j.ecoleng.2017.05.038
Mayer-Pinto, M., Dafforn, K. A., and Johnston, E. L. (2019). A decision framework for coastal infrastructure to optimize biotic resistance and resilience in a changing climate. Bioscience 69, 833–843. doi: 10.1093/biosci/biz092
McAfee, D., Cole, V. J., and Bishop, M. J. (2016). Latitudinal gradients in ecosystem engineering by oysters vary across habitats. Ecology 97, 929–939. doi: 10.1890/15-0651.1
McAfee, D., O’Connor, W. A., and Bishop, M. J. (2017). Fast-growing oysters show reduced capacity to provide a thermal refuge to intertidal biodiversity at high temperatures. J. Anim. Ecol. 86, 1352–1362. doi: 10.1111/1365-2656.12757
Milon, J. W. (1989). Artificial marine habitat characteristics and participation behavior by sport anglers and divers. Bull. Mar. Sci. 44, 853–862.
Morris, R. L., Heery, E. C., Loke, L. H., Lau, E., Strain, E., Airoldi, L., et al. (2019). Design options, implementation issues and evaluating success of ecologically engineered shorelines. Oceanogr. Mar. Biol. 57, 169–228.
Morris, R. L., Konlechner, T. M., Ghisalberti, M., and Swearer, S. E. (2018). From grey to green: efficacy of eco-engineering solutions for nature-based coastal defence. Glob. Change Biol. 24, 1827–1842.1.
Moschella, P. S., Abbiati, M., Åberg, P., Airoldi, L., Anderson, J. M., Bacchiocchi, F., et al. (2005). Low-crested coastal defence structures as artificial habitats for marine life: using ecological criteria in design. Coast. Eng. 52, 1053–1071. doi: 10.1016/j.coastaleng.2005.09.014
Murphy, T. R., Hanley, M. E., Ellis, J. S., and Lunt, P. H. (2019). Deviation between projected and observed precipitation trends greater with altitude. Clim. Res. 79, 77–89. doi: 10.3354/cr01583
Naylor, L., Kippen, H., Coombes, M. A., Horton, B., Macarthur, M., and Jackson, N. (2017). Greening the Grey: a Framework for Integrated Green Grey Infrastructure (IGGI). United Kingdom: University of Glasgow.
Ng, C. S. L., Lim, S. C., Ong, J. Y., Teo, L. M. S., Chou, L. M., and Chua, K. E. et al. (2015). Enhancing the biodiversity of coastal defence structures: transplantation of nursery-reared reef biota onto intertidal seawalls. Ecol. Eng. 82, 480–486. doi: 10.1016/j.ecoleng.2015.05.016
O’Shaughnessy, K. A. (2020). Eco-engineering of coastal infrastructure: a design for life. United Kingdom: University of Plymouth.
O’Shaughnessy, K. A., Hawkins, S. J., Yunnie, A. L., Hanley, M. E., Lunt, P., Thompson, R. C., et al. (2020). Occurrence and assemblage composition of intertidal non-native species may be influenced by shipping patterns and artificial structures. Mar. Pollut. Bull. 154:111082. doi: 10.1016/j.marpolbul.2020.111082
Perkol-Finkel, S., Ferrario, F., Nicotera, V., and Airoldi, L. (2012). Conservation challenges in urban seascapes: promoting the growth of threatened species on coastal infrastructures. J. Appl. Ecol. 49, 1457–1466. doi: 10.1111/j.1365-2664.2012.02204.x
Perkol-Finkel, S., Hadary, T., Rella, A., Shirazi, R., and Sella, I. (2018). Seascape architecture–incorporating ecological considerations in design of coastal and marine infrastructure. Ecol. Eng. 120, 645–654. doi: 10.1016/j.ecoleng.2017.06.051
Perkol-Finkel, S., Zilman, G., Sella, I., Miloh, T., and Benayahu, Y. (2008). Floating and fixed artificial habitats: spatial and temporal patterns of benthic communities in a coral reef environment. Estuar. Coast. Shelf Sci. 77, 491–500. doi: 10.1016/j.ecss.2007.10.005
Peterson, C. H., and Beal, B. F. (1989). Bivalve growth and higher order interactions: importance of density, site, and time. Ecology 70, 1390–1404. doi: 10.2307/1938198
Pickering, H., and Whitmarsh, D. (1997). Artificial reefs and fisheries exploitation: a review of the ‘attraction versus production’ debate, the influence of design and its significance for policy. Fish. Res. 31, 39–59. doi: 10.1016/S0165-7836(97)00019-2
Porri, F., Jordaan, T., and McQuaid, C. D. (2008). Does cannibalism of larvae by adults affect settlement and connectivity of mussel populations? Estuar. Coast. Shelf Sci. 79, 687–693. doi: 10.1016/j.ecss.2008.06.010
Preston, F. (1960). Time and space and the variation of species. Ecology 41, 611–627. doi: 10.2307/1931793
Ramus, A. P., Silliman, B. R., Thomsen, M. S., and Long, Z. T. (2017). An invasive foundation species enhances multifunctionality in a coastal ecosystem. Proc. Natl. Acad. Sci. 114, 8580–8585. doi: 10.1073/pnas.1700353114
Raymond, C. M., Frantzeskaki, N., Kabisch, N., Berry, P., Breil, M., Nita, M. R., et al. (2017). A framework for assessing and implementing the co-benefits of nature-based solutions in urban areas. Environ. Sci. Policy 77, 15–24. doi: 10.1016/j.envsci.2017.07.008
Reise, K. (1998). Pacific oysters invade mussel beds in the European Wadden Sea. Senckenbergiana Mari. 28, 167–175. doi: 10.1007/BF03043147
Scheffers, B. R., Edwards, D. P., Diesmos, A., Williams, S. E., and Evans, T. A. (2014). Microhabitats reduce animal’s exposure to climate extremes. Glob. Chang. Biol. 20, 495–503. doi: 10.1111/gcb.12439
Smith, J. A., Lowry, M. B., Champion, C., and Suthers, I. M. (2016). A designed artificial reef is among the most productive marine fish habitats: new metrics to address ‘production versus attraction’. Mar. Biol. 163, 1–8. doi: 10.1007/s00227-016-2967-y
Sotka, E. E., and Byers, J. E. (2019). Not so fast: promoting invasive species to enhance multifunctionality in a native ecosystem requires strong(er) scrutiny. Biol. Invasions 21, 19–25. doi: 10.1007/s10530-018-1822-0
Steneck, R. S., and Dethier, M. N. (1994). A functional group approach to the structure of algal-dominated communities. Oikos 69, 476–498. doi: 10.2307/3545860
Stolk, P., Markwell, K., and Jenkins, J. M. (2007). Artificial reefs as recreational scuba diving resources: a critical review of research. J. Sustain. Tour. 15, 331–350. doi: 10.2167/jost651.0
Strain, E. M., Olabarria, C., Mayer-Pinto, M., Cumbo, V., Morris, R. L., Bugnot, A. B., et al. (2017a). Eco-engineering urban infrastructure for marine and coastal biodiversity: which interventions have the greatest ecological benefit? J. Appl. Ecol. 55, 426–441. doi: 10.1111/1365-2664.12961
Strain, E. M. A., Morris, R. L., Coleman, R. A., Figueira, W. F., Steinberg, P. D., Johnston, E. L., et al. (2017b). Increasing microhabitat complexity on seawalls can reduce fish predation on native oysters. Ecol. Eng. 120, 637–644. doi: 10.1016/j.ecoleng.2017.05.030
Strain, E. M., Steinberg, P. D., Vozzo, M., Johnston, E. L., Abbiati, M., Aguilera, M. A., et al. (2021). A global analysis of complexity–biodiversity relationships on marine artificial structures. Glob. Ecol. Biogeogr. 30, 140–153. doi: 10.1111/geb.13202
Strain, E. M. A., Cumbo, V. R., Morris, R. L., Steinberg, P. D., and Bishop, M. J. (2020). Interacting effects of habitat structure and seeding with oysters on the intertidal biodiversity of seawalls. PLoS One 15:e0230807.
Striem, H. L. (1967). A comparative study of rainfall frequency-distribution spectra for Jerusalem and Rome. Isr. J. Earth Sci. 16, 22–29.
Temmerman, S., Meire, P., Bouma, T. J., Herman, P. M., Ysebaert, T., and De Vriend, H. J. (2013). Ecosystem-based coastal defence in the face of global change. Nature 504, 79–83. doi: 10.1038/nature12859
Terlizzi, A., Benedetti-Cecchi, L., Bevilacqua, S., Fraschetti, S., Guidetti, P., and Anderson, M. J. (2005). Multivariate and univariate asymmetrical analyses in environmental impact assessment: a case study of Mediterranean subtidal sessile assemblages. Mar. Ecol. Prog. Ser. 289, 27–42. doi: 10.3354/meps289027
Underwood, A. J. (2000). Experimental ecology of rocky intertidal habitats: what are we learning? J. Exp. Mar. Biol. Ecol. 250, 51–76. doi: 10.1016/S0022-0981(00)00179-9
United Nations (UN) (2020). Decade of Restoration. Available online at: https://www.decadeonrestoration.org/ [Accessed 17 January 2021]
Vozzo, M. L., Mayer-Pinto, M., Bishop, M. J., Cumbo, V. R., Bugnot, A. B., Dafforn, K. A., et al. (2021). Making seawalls multifunctional: the positive effects of seeded bivalves and habitat structure on species diversity and filtration rates. Mar. Environ. Res. 165:105243. doi: 10.1016/j.marenvres.2020.105243
Watanuki, N., and Gonzales, B. J. (2006). The potential of artificial reefs as fisheries management tools in developing countries. Bull. Mar. Sci. 78, 9–19.
Whittaker, R. H., Levin, S. A., and Root, R. B. (1973). Niche, habitat, and ecotope. Am. Nat. 107, 321–338. doi: 10.1086/282837
Keywords: urbanization, ocean sprawl, biodiversity, seeding, mussels, artificial structures, eco-engineering, ecological engineering
Citation: O’Shaughnessy KA, Perkol-Finkel S, Strain EMA, Bishop MJ, Hawkins SJ, Hanley ME, Lunt P, Thompson RC, Hadary T, Shirazi R, Yunnie ALE, Amstutz A, Milliet L, Yong CLX and Firth LB (2021) Spatially Variable Effects of Artificially-Created Physical Complexity on Subtidal Benthos. Front. Ecol. Evol. 9:690413. doi: 10.3389/fevo.2021.690413
Received: 02 April 2021; Accepted: 20 July 2021;
Published: 20 August 2021.
Edited by:
Peter M. J. Herman, Delft University of Technology, NetherlandsReviewed by:
Lea T. Mamo, Southern Cross University, AustraliaAlice E. Hall, Bournemouth University, United Kingdom
Copyright © 2021 O’Shaughnessy, Perkol-Finkel, Strain, Bishop, Hawkins, Hanley, Lunt, Thompson, Hadary, Shirazi, Yunnie, Amstutz, Milliet, Yong and Firth. This is an open-access article distributed under the terms of the Creative Commons Attribution License (CC BY). The use, distribution or reproduction in other forums is permitted, provided the original author(s) and the copyright owner(s) are credited and that the original publication in this journal is cited, in accordance with accepted academic practice. No use, distribution or reproduction is permitted which does not comply with these terms.
*Correspondence: Kathryn A. O’Shaughnessy, oshaug3@gmail.com