Habitat Complexity Affects the Structure but Not the Diversity of Sessile Communities on Tropical Coastal Infrastructure
- 1Centre for Marine and Coastal Studies, Universiti Sains Malaysia, Penang, Malaysia
- 2School of Housing, Building and Planning, Universiti Sains Malaysia, Penang, Malaysia
- 3Institute of Biological, Environmental and Rural Sciences, Aberystwyth University, Aberystwyth, United Kingdom
- 4School of Ocean and Earth Science, University of Southampton, National Oceanography Centre Southampton, Southampton, United Kingdom
- 5School of Biological and Marine Sciences, University of Plymouth, Plymouth, United Kingdom
- 6Marine Biological Association of the United Kingdom, The Laboratory, Plymouth, United Kingdom
- 7Institute for Marine and Antarctic Studies, University of Tasmania, Hobart, TAS, Australia
Increasing human population, urbanisation, and climate change have resulted in the proliferation of hard coastal infrastructure such as seawalls and breakwaters. There is increasing impetus to create multifunctional coastal defence structures with the primary function of protecting people and property in addition to providing habitat for marine organisms through eco-engineering - a nature-based solutions approach. In this study, the independent and synergistic effects of physical complexity and seeding with native oysters in promoting diversity and abundances of sessile organisms were assessed at two locations on Penang Island, Malaysia. Concrete tiles with varying physical and biological complexity (flat, 2.5 cm ridges and crevices, and 5 cm ridges and crevices that were seeded or unseeded with oysters) were deployed and monitored over 12 months. The survival of the seeded oysters was not correlated with physical complexity. The addition of physical and biological complexity interacted to promote distinct community assemblages, but did not consistently increase the richness, diversity, or abundances of sessile organisms through time. These results indicate that complexity, whether physical or biological, is only one of many influences on biodiversity on coastal infrastructure. Eco-engineering interventions that have been reported to be effective in other regions may not work as effectively in others due to the highly dynamic conditions in coastal environment. Thus, it is important that other factors such as the local species pools, environmental setting (e.g., wave action), biological factors (e.g., predators), and anthropogenic stressors (e.g., pollution) should also be considered when designing habitat enhancements. Such factors acting individually or synergistically could potentially affect the outcomes of any planned eco-engineering interventions.
Introduction
Climate-change related factors, such as sea level rise and increasing storminess, have led to hardening of shorelines as an adaptive societal response (Adger et al., 2005; Dugan et al., 2011; Gittman et al., 2015). This trend is further exacerbated by the growth in human population and their propensity to live near the coast (Firth et al., 2016a; Gittman et al., 2016). Globally, coastal development schemes of various scales, ranging from the reclamation of coastlines to construction of whole artificial islands, are increasing (Chapman and Bulleri, 2003; Chee et al., 2017; Bugnot et al., 2021). Consequently, natural coastal ecosystems like mangroves, as well as sandy and rocky beaches are rapidly being replaced by artificial structures such as seawalls, breakwaters, and extensive rock revetments (Bishop et al., 2017; Heery et al., 2017; Loke et al., 2019).
While artificial structures serve an important engineering purpose, they have myriad impacts on biodiversity and do not contribute equivalent ecosystem services to the natural habitats they replace (Dafforn et al., 2015; Perkins et al., 2015; Firth et al., 2016a). They alter the physico-chemical (Dafforn et al., 2015; Bishop et al., 2017) and biological (Dugan et al., 2011; Waltham and Sheaves, 2015; Todd et al., 2019) characteristics of the local environment, by modifying hydrodynamics and geomorphology (Dugan et al., 2008; Nordstrom, 2014). They are strong drivers of habitat alteration from soft sediments to hard substrata (Bulleri and Chapman, 2010; Chapman and Underwood, 2011), disrupting ecological connectivity (Firth et al., 2016a; Bishop et al., 2017), thereby altering or accelerating biodiversity loss (Goodsell et al., 2007; Dong et al., 2016) with consequences for ecosystem functioning (Iveša et al., 2010) and supply of ecosystems services (Firth et al., 2016a). Hence, there is an urgent need for strategies to improve the biodiversity and functioning of artificial structures.
Many artificial structures are physically homogeneous and lack the complexity of analogous natural rocky habitats that they often replace (Moschella et al., 2005; Strain et al., 2018; Waltham and Dafforn, 2018; Ushiama et al., 2019) and with this, the diversity of native organisms (Nordstrom, 2014; Heery et al., 2017; Cacabelos et al., 2019). Artificial structures often support only a subset of species from adjacent natural rocky shore assemblages (Chapman, 2003; Firth et al., 2013, 2016b), and can support greater abundances of invasive species (see Mineur et al., 2012 for review) as well as species found less commonly on adjacent rocky shores, such as opportunist early and mid-successional species (Chapman and Bulleri, 2003; Moschella et al., 2005; Evans et al., 2017). To maintain and optimise native biodiversity, ecologically beneficial features that mimic the complexities of natural habitats whilst not compromising structural integrity, need to be incorporated into engineering designs (Moschella et al., 2005; Hoggart et al., 2015; O’Shaughnessy et al., 2020). This can be done through “eco-engineering” interventions—attempts to green grey infrastructure to create multifunctional structures, with a secondary function of providing habitats for marine organisms (Evans et al., 2017, 2019; Naylor et al., 2017; Strain et al., 2018). This nature-based solutions approach has been increasingly applied to restore urban ecosystems and landscapes and to enhance their ecosystem functions and services with different techniques showing varying levels of success (Evans et al., In review).
Pre-cast concrete tiles with physical complexity such as crevices, grooves and/or pits have been attached to surfaces of coastal defence structures in intertidal areas to test their potential to increase species richness and abundance on these structures (see Strain et al., 2018; O’Shaughnessy et al., 2020, 2021 for reviews). These studies have demonstrated that increasing physical complexity can result in greater diversity and abundances of colonising organisms relative to flat surfaces (Strain et al., 2020). Additionally, tiles seeded with native bivalves, thus increasing biotic complexity, can also provide more favourable microhabitats for colonising species than flat surfaces (McAfee et al., 2016; Strain et al., 2020). Seeding foundation species such as bivalves can enhance recruitment of colonising organisms and enable them to reach less vulnerable size classes or developmental stages, through reduction of abiotic stressors (Connell and Slatyer, 1977) and negative biological interactions (Gratwicke and Speight, 2005), and/or by producing faecal matter to serve as food or habitat for infauna (Commito and Boncavage, 1989). They can also deliver critical ecosystem functions such as biofiltration, especially in enclosed port settings such as docks or marinas (Russell et al., 1983; Hughes et al., 2005). Conversely, seeded bivalves may prevent the settlement of other sessile invertebrates and algae via space limitation (Vieira et al., 2018), filtration of settling larvae (André et al., 1993), and/or competition for food (Commito and Boncavage, 1989).
To date, most eco-engineering studies have tested the effects of adding physical and biological complexity separately (but see Bradford et al., 2020; Strain et al., 2020; Vozzo et al., 2021). The positive effects of physical and biological complexity for biodiversity may be enhanced by combining the two approaches (Strain et al., 2018; Bradford et al., 2020; Vozzo et al., 2021). In a study where complexity was manipulated through habitat structure and seeding with native bivalves on concrete tiles attached onto seawalls, greater richness and diversity of taxa and higher abundance of suspension feeding taxa were recorded on physically complex tiles seeded with bivalves compared to tiles with no complexity or unseeded tiles (Vozzo et al., 2021). Greater species density of sessile taxa has also been reported for complex seeded tiles relative to flat unseeded tiles (Strain et al., 2020). To date most of this research has, however, been undertaken in temperate locations (Strain et al., 2018). Proof-of-concept trials from tropical regions, where the effects of temperature and desiccation are more pronounced and of greater threat to intertidal organisms, are lacking (but see Loke et al., 2019; Bradford et al., 2020; Chee et al., 2020). The World Harbour Project (WHP) was designed to address this knowledge gap, by deploying complex tiles manufactured using 3D printing technology to mimic natural habitat features on rocky shores on seawalls, globally (Strain et al., 2021).
Penang, Malaysia, was one of the locations where the global WHP experiment was carried out. It is the only harbour in the experiment that is located in the tropics. The coastline of Penang is heavily developed, with extensive land reclamation along the coast and building of artificial islands (Chee et al., 2017). The seawalls and rock revetments which dominated the coastline area colonised by barnacles and green algae with sparser oysters, tunicates, and polychaetes (Chee, personal observation). We investigated the interactive effects of increasing physical and biological habitat complexity on biodiversity by adding tiles with ridges and crevices (flat, 2.5 cm, 5 cm, and controls) combined with oyster-seeding (Magallana bilineata Röding, 1798), onto seawalls in two locations (Penang Port and Straits Quay Marina). More specifically, we tested the effectiveness of complexity in increasing taxon richness, diversity, and the abundance of colonising sessile organisms. We hypothesised that the addition of physical complexity would: (1) increase the survival of seeded oysters by protecting them from large-bodied predators; (2) enhance the richness, diversity, abundance, and community structure of sessile organisms by providing cool and moist crevice habitats (Strain et al., 2020); (3) have synergistic effects with biological complexity on the richness, abundance, diversity, and community structure of colonising benthic organisms (Strain et al., 2020). The results from our study, would then be used to inform future eco-engineering approaches for coastal structures in Malaysia and elsewhere in the tropics.
Materials and Methods
Site Description
The experiment was conducted at Penang Port (5°24′57″ N, 100°20′38″ E) and Straits Quay Marina (5°27′34″ N, 100°18′52″ E) (Figure 1). Both sites have semi-diurnal tidal regimes, with tidal range between 0 and 3.0 m. Penang Port, which is one of the busiest ports in the world, is protected by concrete seawalls. Water quality in this area is poor, contaminated with organic material, suspended solids, oil, and grease plus sewage indicated by the presence of Escherichia coli (Shin and Abllah, 2015). Straits Quay Marina is protected by two concrete breakwaters. Sedimentation is relatively high within the marina and periodic dredging is required. Wild populations of oysters occur naturally at both sites. In Penang Port, oysters were densely distributed (160 oysters/m2) on the mid tidal level whilst in Straits Quay Marina, oysters occurred in patches along the mid tidal level and in higher densities lower down. Sessile organisms found naturally occurring on coastal artificial structures in the vicinity include barnacles Amphibalanus amphitrite and Chthamalus malayensis, oysters Saccostrea cucullata, mussels Perna viridis (Chee et al., 2020), and on adjacent natural rocky shores the barnacles, oysters, and sea anemone Anthopleura dixoniana and Anthopleura nigrescens, were commonly found.
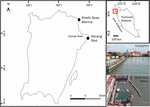
Figure 1. Map of Penang Island, Malaysia, showing experimental sites, Straits Quay Marina and Penang Port.
Experimental Design
At each site, five replicates of seeded and unseeded flat, 2.5 cm, and 5 cm concrete tiles measuring 0.25 × 0.25 m were deployed to test our hypotheses about the effects of physical and biological complexity on colonising communities (Supplementary Figure 1). The concrete tiles were deployed at 0.7 m above mid tide level within a tidal range of 0.3–3.0 m (tidal range measured at tide gauge station located in Kedah Pier, Penang). The concrete tiles were designed and manufactured by Reef Design Lab, Australia, using 3D printing technology. These had textured surfaces from the 3D printing process and three levels of physical complexity: low, intermediate, and high (Strain et al., 2018). The flat tiles did not have ridges or crevices; these had a total surface area of 0.06 m2. The intermediate complexity tiles had 2.5 cm high ridges separated by 1.5–5 cm wide crevices and a total surface area of 0.09 m2. The most complex tiles had 5 cm high ridges separated by 1.5–5 cm wide crevices and had total surface area of 0.14 m2.
Slipper oysters, M. bilineata, which can be found naturally on seawalls at both sites, were transplanted onto half of the low, intermediate, and high complexity tiles. Juvenile oysters (measuring ∼3 cm in length) were sourced from a local aquaculture farm and glued (DEVTON 2 Ton Epoxy-Clear) to the tiles in groups of four individuals to give approximately 35% cover on each tile. The epoxy glue was chosen as an adhesive because of its high strength, low shrinkage, excellent adhesion to various substrates, low cost, and low toxicity. On 2.5 and 5 cm complex tiles, half the number of oysters were glued onto the ridges and the other half to the crevices. On flat tiles, four groups were glued in a standardised pattern in the four corners. Five additional control tiles were deployed for comparison at each site. These were flat and produced locally from the same material as the concrete seawall/breakwater. Tiles were attached to aluminium frames in a randomised order in a single horizontal row, with crevices and ridges orientated vertically. The aluminium frames were suspended from the seawall/breakwater at mid-tidal level, facing the open sea.
Seeded Oyster Survivorship
After 1, 3, 6, 9, and 12 months, the survival of the seeded oysters was assessed in situ during low tide, by counting the number of live individuals remaining on the tiles and for complex tiles in the either the crevice or ridge microhabitats. Missing oysters were recorded as “dislodged.” Oysters with closed shells were considered alive and those with shells that were open, or empty were considered dead. The dominant source of mortality was inferred qualitatively by examining the dead oyster shells for signs of damage by predators (e.g., scratch marks from monitor lizards, drill holes from gastropods) and comparing with environmental parameters (e.g., pollution and/or extreme weather events). “Cracked” was interpreted as mortality from physical damage, either from predation, boats, or floating debris; and “intact” was considered as mainly mortality from environmental stressors such as pollution or extreme temperatures, although disease or senescence cannot be ruled out.
Colonising Taxa
At 1, 3, 6, 9, and 12 months, all the tiles were photographed in situ with a photo quadrat frame at a fixed distance (0.5 m perpendicular to tiles) using a GoPro Hero5. The images were processed using PhotoQuad (Trygonis and Sini, 2012) to assess the abundance in percentage cover of algae and sessile animals by overlaying a grid of 100 random points. On complex tiles, 50 points were laid on ridges and 50 on crevices. Taxa directly under the points were counted. When in situ identifications were not possible, samples of taxa were retrieved and identified to species or generic level (but in the case of serpulids and didemnids to family level) (Lim et al., 2009; Taylor and Tan, 2015), with the aid of a stereo microscope (ZEISS Stemi 305) when required. The seeded oysters were excluded from the abundance estimates. Mobile species were not recorded because they were not always visible in the photographs.
Data Analyses
The effects of physical complexity (fixed: flat, 2.5 cm, 5 cm), time (fixed repeated measures: 1, 3, 6, 9, 12 months), and site (random: Penang Port, Straits Quay Marina) on the survival of seeded oysters were tested with Linear Mixed-effect Model (LMM). Where a significant difference was detected, Tukey’s HSD comparisons were used to explore sources of treatment variation. Additionally, the effects of the microhabitats (fixed: crevices, ridges) on survival of seeded oysters was assessed.
To test the hypotheses about the effects of physical complexity (fixed: flat, 2.5 cm, 5 cm, controls), bivalve seeding (covariate: number of live oysters), time (fixed repeated measures: 1, 3, 6, 9, 12 months), and site (random: Penang Port, Straits Quay Marina) on the taxon richness, Shannon’s diversity index and abundance (total live cover) of macroalgae and sessile invertebrate assemblages on the tiles, generalised linear mixed model (GLMM) was used to analyse each model, respectively. Due to high rates of bivalve mortality throughout the duration of this the experiment, we also included the surviving seeded bivalves as a covariate factor instead of main factor in the analyses. All richness, diversity, and abundance were modelled using Poisson distribution with log link. P-values were calculated by Wald chi-squared test on all factors presented in each model. Where appropriate post hoc comparisons were run with the “emmeans” package (Lenth, 2020).
To test the effects of physical complexity (as above), seeding (covariate: as above), time (fixed repeated measures: as above), and site (random: as above), on the sessile community assemblages on tiles we used PERMANOVA using the “vegan” community ecology package version 2.5–5 (Oksanen et al., 2014) and “pairwiseAdonis” pairwise multilevel comparison (Martinez Arbizu, 2020). Species–abundance data were analysed using Bray-Curtis distance and square-root transformed to meet the assumptions of normality. The analysis was visualised using nMDS plots. All statistical analyses were done using R (Version 4.0).
Results
Seeded Oyster Survivorship
At both sites, the percentage of live seeded oysters on tiles declined through time, and after 12 months, average survival was <10% on flat and complex tiles (Figure 2). There was no significant difference in oyster survivorship among complexities nor sites (Tables 1, 2). Regardless of complexity treatment, the main source of mortality across both sites appeared to be from dislodgement from tiles (Figure 3). Despite of this, we found a significant difference on oyster survival between crevices and ridges (Table 2). On average, seeded oysters had higher chances of survival on the crevices than in the ridges, regardless of treatment type. After 12 months, the average cover of seeded oyster in crevices and ridges were 3.74 and 1.96%, respectively.
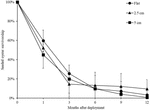
Figure 2. Mean (±SE) percentage of seeded oyster survivorship on seeded flat, 2.5 and 5 cm tiles through time, averaged across both sites. Survival at each time point was in relation to the original seeded population.
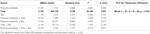
Table 1. Linear mixed effect model testing the effects of physical complexity and time on seeded oyster survivorship withsite as random factor.
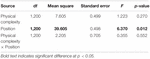
Table 2. Linear mixed effect modal comparing seeded oyster survivorship between crevices and ridges (position) of 2.5 and 5 cm complex tiles.
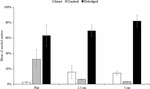
Figure 3. Mean (±SE) percentage of dead seeded oysters recorded as intact, cracked, or dislodged from seeded flat, 2.5 and 5 cm tiles deployed at both sites. Data were pooled over the five surveys (1, 3, 6, 9, and 12 months).
Taxa Richness, Shannon’s Diversity, and Abundance
In total, 20 taxa were recorded on tiles across both sites (Supplementary Table 1). All taxa were native to Malaysia except for the bryozoan Bugula neritina and the oyster Magallana gigas, which are classified as invasive (Taylor and Tan, 2015; Lim et al., 2017). Eight taxa were found on all treatments and control tiles at both sites, including the barnacles A. amphitrite and Amphibalanus reticulatus, newly recruited oysters M. bilineata and S. cucullata (0.5–1.0 cm), serpulid worms, didemnid ascidians, and algae Bryopsis sp. and turf algae. Three species (the barnacle Tetraclita squamosa, oyster Saccostrea mordax, and mussel P. viridis), were only recorded on complex tiles (both 2.5 and 5 cm), while the mussel Xenostrobus sp. was found on 5 cm tiles only.
The effect of physical complexity on the taxa richness of colonising macroalgae and sessile invertebrates varied through time (Table 3). Mean richness was significantly higher on the 2.5 cm tiles than the flat tiles, but only at month 1. Thereafter, there was no significant effect of physical complexity on richness, regardless of seeding treatment (Figure 4A and Supplementary Table 2). Similarly, there was no significant effect of physical nor biological complexity (i.e., the number of live seeded bivalves remaining on tiles) on diversity over time (Table 3). Diversity was slightly higher on complex tiles than control tiles (but not flat) after month 1, but higher on controls than other treatments at month 3, but not significantly different between any treatments for the remainder of the study (Figure 4B). Across all time points combined, more taxa accumulated on the complex tiles than the flat or control tiles (5 cm > 2.5 cm > flat = control tiles) (Figure 5).
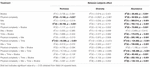
Table 3. Summary of generalised linear mixed model on the effects of physical complexity (flat, 2.5 cm, 5 cm, control) and the number of live seeded bivalves on the taxa richness, Shannon’s diversity, and abundance (total percentage of live cover) of colonising sessile organisms through time, with site as random factor.
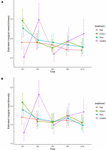
Figure 4. Effects of physical complexity (pooled over unseeded and seeded treatments at both sites) on the (A) mean taxa richness and (B) mean diversity (Shannon index) of the recruited sessile organisms at each time point. Note that the lower and upper errors are absolute values.
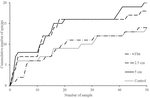
Figure 5. Cumulative number of taxa on flat, 2.5 cm, 5 cm, and control tiles at both sites through time.
In contrast, the interaction between physical and biological complexity significantly affected the abundance of organisms, but this effect also varied over time and site (Table 3). Generally, the percentage cover of sessile organisms at Penang Port was highest on the most complex tiles 5 cm, followed by 2.5 cm, flat, and control tiles, but these differences in percentage cover were non-significant (Figure 6A and Supplementary Table 3). At Straits Quay Marina, the control had significant higher percentage cover of the colonising sessile organisms, than the other treatments, but this results was not consistent through time (Figure 6B and Supplementary Table 3).
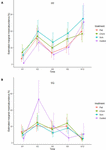
Figure 6. Effects of physical complexity (pooled over unseeded and seeded treatments) on the mean abundances (total percentage of live cover) of the recruited sessile organisms at two study sites: (A) PP: Penang Port and (B) SQ: Straits Quay Marina, Penang Malaysia, at each time point.
Sessile Community Assemblage
The sessile community assemblage was significantly affected by the interactions between physical complexity, site, and time; physical complexity, the number of live seeded bivalves, and site; and physical complexity, the number of seeded bivalves, and time (Table 4 and Supplementary Figure 2). When the seeded and unseeded treatments were pooled, the post hoc comparisons showed that there were significant differences in the benthic assemblages between the complex tiles (i.e., 2.5 and 5 cm) and the controls (but not the flat tiles), at all-time points excluding month 9 at Penang Port (Table 5). The taxa that contributed to the significant differences were oyster M. sikamae and barnacle T. squamosa which were both found in higher abundances on complex compared to control tiles. At Straits Quay Marina, there were also significant differences between the complex and control tiles (but not flat), at all time-points except for month 1 (Table 5). The taxa that contributed to the significant differences at this site were green-lipped mussel P. viridis and Xenostrobus sp. which were present exclusively on complex tiles.
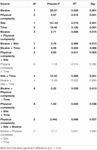
Table 4. PERMANOVA testing the effect of physical complexity, number of live seeded bivalves, and time on the colonising sessile assemblages at two sites.
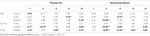
Table 5. Results of Tukey pairwise comparisons testing the effects of physical complexity (pooled over seeded and unseeded treatments) on the recruited sessile assemblage at each site at each time point.
When biological complexity (number of surviving oysters) was considered together with physical complexity, the sessile assemblages at both sites responded differently through time. Significant differences in sessile assemblages at both sites were detected in the earlier months of the experiment but not after 9 months (Table 6).
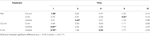
Table 6. Results of Tukey pairwise comparison testing the effects of physical complexity on the recruited sessile assemblage at each time point with the number of live seeded bivalves as covariates.
At Penang Port, the assemblages were significantly different between flat and 5 cm tiles only but, at Straits Quay Marina, assemblages on control tiles were significantly different with all the other complexities (i.e., flat, 2.5 cm, and 5 cm) (Table 7). The taxa that contributed to the significant differences at Penang Port were T. squamosa, Hyotissa inermis, and S. scyphophilla which were found on 5 cm complex tiles. In Straits Quay Marina, the significant differences were contributed by low numbers of A. amphitrite and didemnids and higher numbers of M. bilineata, S. cuccullata, and B. neritina, on control tiles.
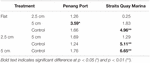
Table 7. Results of Tukey pairwise comparison testing the effects of physical complexity on the recruited sessile assemblage at each site with the number of live seeded bivalves as covariates.
Discussion
Our study provides the first experimental test of how the effects of adding physical habitat complexity (crevices and ridges) and seeding with habitat-forming species (M. bilineata) affects the diversity of sessile organisms on artificial structures in a tropical, urban marine environment, through time. We found that the addition of physical complexity did not increase the survival of seeded oysters and that neither physical complexity alone nor its synergism with biological complexity did not necessarily have consistent benefits for the biodiversity of macrobenthic organisms. These results contradicted with the outcomes of Vozzo et al. (2021) and Strain et al. (2020) where the addition of complexity, and surface area through habitat structure and seeding increased species richness and diversity of sessile taxa in Sydney. Conversely, Strain et al. (2021) found negative or no effects of adding physical complexity and surface area on diversity of sessile taxa in 3 locations, across the globe (see also O’Shaughnessy et al., 2021 which was conducted in the subtidal). This suggests that the effects of complexity on the diversity of colonising organisms is context dependent and varies between locations.
Effect of Physical Complexity on the Survival of Seeded Oysters
We found the addition of physical complexity did not increase the survival of the seeded oysters. This finding was different to the results from Strain et al. (2020) and Vozzo et al. (2021) where over half of the seeded oysters, remained on the tiles affixed to sandstone seawalls in Sydney Harbour. Most of the seeded M. bilineata were dislodged from the tiles in this study (81% in Penang Port and 71% in Straits Quay Marina). This could be due to glue failure but when seeded oysters in crevices and ridges were compared, seeded oysters in crevices had higher percentage cover (3.74 in crevices compared to 1.96% on ridges) indicating that other external factors could have also contributed to the dislodgement. As epoxy (a strong adhesive) was used and residues of it were observed after dislodgement, we suggest that the dislodgement was more likely caused by the marine debris and natural predators which were highly abundant in the two sites in Penang. Large rafts of debris comprising logs, ropes and plastic bottles have been observed hitting against the breakwaters. The impact from collision between large-sized/voluminous marine debris against the tiles could have caused dislodgement of oysters from the tiles. There is also possibility that the oysters were clawed by monitor lizards from the tiles as reported by members of the public and local coastal community living in the vicinity of the study locations.
Previous studies have reported that physical complexity can have favourable outcomes for bivalve survival (Bertolini et al., 2018; Strain et al., 2018, 2020) which in turn results in enhanced biodiversity of colonising organisms (Ysebaert et al., 2019). Allowing bivalves to naturally recruit on the tiles in a mesocosm setting before they are placed in the field, could help to prevent the bivalves from being dislodged. Although comparisons between adhesive strengths via epoxy and natural recruitment has never been tested, oysters that naturally recruit onto hard substrata have been reported to have high adhesive strengths (Kavanagh et al., 2001). Fencing could also be set up to protect transplants against dislodgement through wave action, collisions with floating debris and predation (Ferrario et al., 2016; Schotanus et al., 2020).
Effect of Physical Complexity on Biodiversity and Community Structure
In the global study carried out by Strain et al. (2021), the addition of physical complexity consistently enhanced sessile invertebrate species richness in most (11 out of 14 locations) of the study sites regardless of their regional climates. However, our study in Penang showed no differences in the species richness of sessile organisms between complex and flat tiles. This could be because the type of complexity on the tiles was not suitable habitat for local benthic organisms within the study sites in Penang. Indeed, the use of new introductory microhabitats largely depends on the native species pool and how these species choose habitats. In previous studies, it was found that organisms tend to select for microhabitats similar to their body size (Englund and Cooper, 2003; McAbendroth et al., 2005; Yeager and Hovel, 2017). Bigger organisms would choose larger living spaces, with smaller organisms selecting smaller living spaces (Chapman and Clynick, 2006; Strain et al., 2018). Some organisms even select for colour when choosing habitats (Satheesh and Wesley, 2010; Dobretsov et al., 2013). The amount of shade, light, and moisture provided by the microhabitats are critical factors in habitat selection (Firth et al., 2016b; Cordell et al., 2017; Strain et al., 2017; Amstutz et al., 2021). Besides the physical attributes, the chemical properties of the surrounding waters (e.g., pH, pollutants, heavy metal) could have also prevented the recruitment of additional species onto the complex and flat tiles. Additional experiments on the relationship between the size of organisms and microhabitat complexity should be carried out to elucidate the specific cause of the disparity. However, it is also clear that consideration of environmental factors is crucial when designing suitable habitats for marine life on coastal infrastructure.
It is also worth noting that, although not significantly different, cumulative species richness was highest on the complex tiles compared to the flat and control tiles suggesting that physical complexity may have had a slight degree of influence on the number species recruiting on these tiles over time. However, there are some caveats with regards to this including (1) species may recruit and die within a short time and could still contribute to the higher cumulative number of species on the complex tiles, and (2) complex tiles have larger areas which may result in a higher cumulative number of species. Larger areas can provide more space for organism colonisation which could then contribute to higher cumulative species richness. Nevertheless, this study, which manipulated a single type of habitat complexity (crevices/ridges), was not designed to disentangle complexity effects arising from enhancement of surface area and microhabitat diversity.
In terms of abundance, we also found no significant differences between physically complex tiles and flat and control tiles. The complex tiles may not have protected the recruiting organisms from small-bodied predators in Penang. Even if complex habitats can influence predator-prey interactions, small-bodied predators could also benefit from the complexity, leading to increased predation rates in complex habitats (Naylor and McShane, 1997). Our results also indicate that the complex tiles may not have provided adequate shelter from harsh conditions by providing conducive shade and temperature which is known to affect abundance positively (Blockley and Chapman, 2006; Chapman and Blockley, 2009; Firth et al., 2016b). Thus, we emphasise that case-specific designs that take into consideration the species pool and interactions, plus environmental context, are essential to increase the likelihood of desired outcomes.
Although physical complexity did not significantly affect species richness, abundance, and diversity, it did result in significantly different community structures. Generally, at both sites in this study, higher complexities were found to have a different community structure from intermediate and low complexities, as well as the controls. The lack of microhabitats (created by ridges and crevices) on flat and control tiles could have resulted in high inter- and intraspecific competition in terms of space and availability of food (Kovalenko et al., 2012). As for the differences in community structure found between flat and control tiles that both lack complexity, the type of materials used could have contributed to this finding. Indeed, native species richness and composition have been reported to be affected by concrete type (McManus et al., 2018) and ecologically friendly material have greater biodiversity of colonising taxa (Dennis et al., 2018). Importantly, our study focused on sessile benthic communities (macroalgae and sessile invertebrates) but not mobile species. Previous studies have shown that added microhabitat complexity can promote the diversity and abundance of mobile invertebrates on structure surfaces (Evans et al., 2016; Hall et al., 2019; Strain et al., 2020) and influence benthic fish behaviour on and around surfaces (Morris et al., 2017; Ushiama et al., 2019; Strain et al., 2020). Our study indicates that these effects may be directly driven by the physical refuge provided by complex surfaces, rather than indirectly through interactions with colonising sessile species (but see Taira et al., 2020).
Importantly, the species composition on the complex tiles is different from the species found naturally occurring in the vicinity. Species commonly found in the lower regions of the intertidal area of coastal structures and natural rocky shores such as the didemnid ascidians can be found on complex tiles which were placed in the middle regions. Although present in smaller numbers, A. reticulatus, Striatobalanus tenuis, T. squamosa, H. inermis, and S. mordax which are species that are not commonly found on nearby coastal artificial structures, were also found on the complex tiles. Niches introduced by the tiles seemed to have provided habitat for a new range of species which would otherwise not have survived in the harsh environments present on the artificial structures. This indicates that the tiles did promote the number and percentage cover of certain species but the differences were not sufficient to result in any significant difference. The time frame of the experiment could have reduced the number of species recruiting to the tiles.
At least one invasive bryozoan, B. neritina, was found on the tiles at Straits Quay Marina. Another possibly invasive species, M. gigas (see Wells et al., 2019), was found on all tiles in both sites. Importantly, their distribution in the tidal range was observed to extend higher from low- to mid-tide level on tiles; a level at which they are rarely found on quay walls or natural substrata. Indeed, introduction of novel habitats has been reported to encourage colonisation of non-native species due to their opportunistic and hardy traits of species (Airoldi et al., 2015), compared to the sensitivity and habitat specificity of native species (Miller and Etter, 2008; Dafforn et al., 2009). This demonstrates the importance of understanding the native species pool and their habitat preferences to inform future integrated greening-of-grey infrastructure efforts in this and other tropical regions.
Synergistic Effect of Physical and Biological Complexity on Species Richness, Abundance, and Diversity
Most of the seeded oysters died during the course of the experiment and therefore, did not provide enough biological complexity to significantly influence the diversity. However, recruited oysters were found to have colonised the tiles despite the death of most of the seeded oysters. Although dead, it should not be discounted that the remnant shells from the intact and cracked seeded oysters may have influenced the recruitment of the oysters from the wild either through chemical signals which has previously been reported in Hadfield and Paul (2001) and Carroll et al. (2015), or through residual complexities provided by the remaining shells. Nevertheless, these effects were not explored in this study and whether the small amount of remnant shells from the intact and cracked seeded oysters could have influenced the recruitment of oysters on artificial substrata, remains a hypothesis.
We hypothesised that increasing habitat complexity through adding physical structure and using habitat-forming organisms such as oysters could increase species richness and diversity by introducing biogenic surface for attachment (McAfee et al., 2018; Strain et al., 2020; Firth et al., 2021; Vozzo et al., 2021). However, in this experiment, the synergistic effect of adding physical complexity through the crevices and ridges of the complex tiles and the biological complexity through seeding with oysters, did not increase the diversity of the colonising organisms. There was no significant difference in the species richness, abundance, and diversity between the seeded tiles and the unseeded tiles suggesting that the addition of biological complexity in the form of native oysters did not affect early settlement patterns. Interestingly, in terms of seeded tiles, the higher the survival rate of seeded oysters, the lower the diversity index. The addition of oysters on the tiles seemed to have limited the space available for settlement of other sessile organisms. Furthermore, filter feeders are known to remove larvae of other species from the water column (Porri et al., 2008), which could have resulted in lower recruitment to the seeded tiles hence, lowering the diversity. Our results suggest that the synergy of both physical complexity and seeding with oysters have a limited influence on the biodiversity of coastal infrastructure, and interaction of adding physical and biological complexities may not necessarily enhance outcomes. In some settings they could even cancel each other out. In an eco-engineering application, this could result in inefficient use of resources, time, and labour.
Conclusion
We studied the effects of physical (precast tiles with crevices/ridges) and biological complexity (through seeded oysters) on the species richness, diversity, abundance, and community structure of colonising macrobenthos in Penang, Malaysia. We showed that the addition of physical complexity promoted distinct community assemblages, but did not consistently increase the richness, diversity, or abundances of sessile organisms through time, and little was gained by the additional seeding of oysters. The findings of this study highlighted the effects of site and time, as expected from extremely heterogeneous coastal ecosystems. The main effects were site-specific demonstrating that strategies that have been shown to be effective in other geographical locations may not applicable under every circumstance and local conditions can significantly affect the outcomes. In addition, the lack of effect for most of the variables when testing biological complexity between present study and previous studies could be caused by the difference in the percentage of remaining seeded oysters (∼5% in the current study compared to ∼50% in previous studies). Reporting such unintended outcomes from eco-engineering trials is essential as it informs the improvement of future designs (Firth et al., 2020). Nature-based solutions approaches such as this should take into consideration the local species pools as well as identify local biological (e.g., predators) and physicochemical stressors (e.g., pollution) and disturbance (wave energy made worse by floating debris) before designing the habitat enhancements and when selecting a species to be used for seeding, in future applications to achieve desirable outcomes.
Data Availability Statement
The raw data supporting the conclusions of this article will be made available by the authors, without undue reservation.
Author Contributions
SYC led the World Harbour Project in Penang. JCY was involved in the project set-up, data collection, and data analyses. CBC was involved in construction, installation, and monitoring of the control tiles. AE was involved with the project set-up and data analyses. LF and SH sponsored the World Harbour Project tiles in Penang. ES provided experimental protocols and was involved in the data analyses. All authors contributed to the writing of this manuscript.
Funding
This study was supported financially by The Rufford Foundation (Project no.: 20933-2), E&O Berhad, E&O Property (Penang) Sdn. Bhd., Macro Dimension Concrete Sdn. Bhd., Universiti Sains Malaysia Research University grants (Grant no.: 304/PPANTAI/650876/E117 and 304/PPANTAI/650827/E118), the Royal Society International Exchanges Grant (IE150435), the British Ecological Society Small Grant (5546-6590), the Ian Potter Foundation, Harding Miller Foundation, and The New South Wales Government Office of Science and Research. AE was supported by the Ecostructure project, which is part-funded by the European Regional Development Fund (ERDF) through the Ireland Wales Cooperation Programme 2014–2022.
Conflict of Interest
The authors declare that the research was conducted in the absence of any commercial or financial relationships that could be construed as a potential conflict of interest.
Publisher’s Note
All claims expressed in this article are solely those of the authors and do not necessarily represent those of their affiliated organizations, or those of the publisher, the editors and the reviewers. Any product that may be evaluated in this article, or claim that may be made by its manufacturer, is not guaranteed or endorsed by the publisher.
Acknowledgments
We thank the staff, students, volunteers, and industrial trainees at the Centre for Marine and Coastal Studies and School of Housing Building and Planning, for their contributions in field work and supporting SDG14-Life Below Water. Equipment and facilities required for this project are from the Centre for Marine and Coastal Studies. We also thank Reef Design Lab Pty Ltd for designing and producing the tiles used during the study.
Supplementary Material
The Supplementary Material for this article can be found online at: https://www.frontiersin.org/articles/10.3389/fevo.2021.673227/full#supplementary-material
References
Adger, W. N., Hughes, T. P., Folke, C., Carpenter, S. R., and Rockström, J. (2005). Social-ecological resilience to coastal disasters. Science 309, 1036–1039. doi: 10.1126/science.1112122
Airoldi, L., Turon, X., Perkol-Finkel, S., and Rius, M. (2015). Corridors for aliens but not for natives: effects of marine urban sprawl at a regional scale. Divers. Distrib. 21, 755–768. doi: 10.1111/ddi.12301
Amstutz, A., Firth, L. B., Spicer, J. I., and Hanley, M. E. (2021). Facing up to climate change: temperature variation and the influence of aspect in the rocky intertidal. Mar. Environ. Res. (In Press).
André, C., Jonsson, P. R., and Lindegarth, M. (1993). Predation on settling bivalve larvae by benthic suspension feeders: the role of hydrodynamics and larval behaviour. Mar. Ecol. Prog. Ser. 97, 183–192.
Bertolini, C., Montgomery, W. I., and O’Connor, N. E. (2018). Habitat with small inter-structural spaces promotes mussel survival and reef generation. Mar. Biol. 165:163. doi: 10.1007/s00227-018-3426-8
Bishop, M. J., Mayer-Pinto, M., Airoldi, L., Firth, L. B., Morris, R. L., Loke, L. H., et al. (2017). Effects of ocean sprawl on ecological connectivity: impacts and solutions. J. Exp. Mar. Biol. Ecol. 492, 7–30. doi: 10.1016/j.jembe.2017.01.021
Blockley, D. J., and Chapman, M. G. (2006). Recruitment determines differences between assemblages on shaded or unshaded seawalls. Mar. Ecol. Prog. Ser. 327, 27–36. doi: 10.3354/meps327027
Bradford, T. E., Astudillo, J. C., Lau, E. T., Perkins, M. J., Lo, C. C., Li, T. C., et al. (2020). Provision of refugia and seeding with native bivalves can enhance biodiversity on vertical seawalls. Mar. Pollut. Bull. 160:111578. doi: 10.1016/j.marpolbul.2020.111578
Bugnot, A. B., Mayer-Pinto, M., Airoldi, L., Heery, E. C., Johnston, E. L., Critchley, L. P., et al. (2021). Current and projected global extent of marine built structures. Nat. Sustain. 4, 33–41. doi: 10.1038/s41893-020-00595-1
Bulleri, F., and Chapman, M. G. (2010). The introduction of coastal infrastructure as a driver of change in marine environments. J. Appl. Ecol. 47, 26–35. doi: 10.1111/j.1365-2664.2009.01751.x
Cacabelos, E., Thompson, R. C., Prestes, A. C., Azevedo, J. M. N., Neto, A. I., and Martins, G. M. (2019). Patchiness in habitat distribution can enhance biological diversity of coastal engineering structures. Aquat. Conserv. 29, 127–135. doi: 10.1002/aqc.2972
Carroll, J. M., Riddle, K., Woods, K. E., and Finelli, C. M. (2015). Recruitment of the eastern oyster, Crassostrea virginica, in response to settlement cues and predation in North Carolina. J. Exp. Mar. Biol. Ecol. 463, 1–7.
Chapman, M. G. (2003). Paucity of mobile species on constructed seawalls: effects of urbanization on biodiversity. Mar. Ecol. Prog. Ser. 264, 21–29. doi: 10.3354/meps26402
Chapman, M. G., and Blockley, D. J. (2009). Engineering novel habitats on urban infrastructure to increase intertidal biodiversity. Oecologia 161, 625–635. doi: 10.1007/s00442-009-1393-y
Chapman, M. G., and Bulleri, F. (2003). Intertidal seawalls—new features of landscape in intertidal environments. Landsc. Urban Plan. 62, 159–172. doi: 10.1016/S0169-2046(02)00148-2
Chapman, M. G., and Clynick, B. G. (2006). Experiments testing the use of waste material in estuaries as habitat for subtidal organisms. J. Exp. Mar. Biol. Ecol. 338, 164–178. doi: 10.1016/j.jembe.2006.06.018
Chapman, M. G., and Underwood, A. J. (2011). Evaluation of ecological engineering of “armoured” shorelines to improve their value as habitat. J. Exp. Mar. Biol. Ecol. 400, 302–313. doi: 10.1016/j.jembe.2011.02.025
Chee, S. Y., Othman, A. G., Sim, Y. K., Adam, A. N. M., and Firth, L. B. (2017). Land reclamation and artificial islands: walking the tightrope between development and conservation. Glob. Ecol. Conserv. 12, 80–95. doi: 10.1016/j.gecco.2017.08.005
Chee, S. Y., Wee, J. L. S., Wong, C., Yee, J. C., Yusup, Y., and Mujahid, A. (2020). Drill-Cored Artificial Rock Pools Can Promote Biodiversity and Enhance Community Structure on Coastal Rock Revetments at Reclaimed Coastlines of Penang, Malaysia. Trop. Conserv. Sci. 13:1940082920951912. doi: 10.1177/1940082920951912
Commito, J. A., and Boncavage, E. M. (1989). Suspension-feeders and coexisting infauna: an enhancement counter example. J. Exp. Mar. Biol. Ecol. 125:33. doi: 10.1016/0022-0981(89)90214-1
Connell, J. H., and Slatyer, R. O. (1977). Mechanisms of succession in natural communities and their role in community stability and organization. Am. Nat. 11, 1119–1144.
Cordell, J. R., Toft, J. D., Munsch, S. H., and Goff, M. (2017). “Benches, beaches, and bumps: how habitat monitoring and experimental science can inform urban seawall design,” in Living shorelines: the science and management of nature-based coastal protection, eds D. M. Bilkovic, M. M. Mitchell, M. K. La Peyre, and J. D. Toft (Boca Raton: CRC Press), 421–438.
Dafforn, K. A., Glasby, T. M., Airoldi, L., Rivero, N. K., Mayer-Pinto, M., and Johnston, E. L. (2015). Marine urbanization: an ecological framework for designing multifunctional artificial structures. Front. Ecol. Environ. 13, 82–90. doi: 10.1890/140050
Dafforn, K. A., Johnston, E. L., and Glasby, T. M. (2009). Shallow moving structures promote marine invader dominance. Biofouling 25, 277–287. doi: 10.1080/08927010802710618
Dennis, H. D., Evans, A. J., Banner, A. J., and Moore, P. J. (2018). Reefcrete: reducing the environmental footprint of concretes for eco-engineering marine strcutures. Ecol. Eng. 120, 668–678.
Dobretsov, S., Abed, R. M., and Voolstra, C. R. (2013). The effect of surface colour on the formation of marine micro and macrofouling communities. Biofouling 29, 617–627. doi: 10.1080/08927014.2013.784279
Dong, Y. W., Huang, X. W., Wang, W., Li, Y., and Wang, J. (2016). The marine ‘great wall’ of China: local-and broad-scale ecological impacts of coastal infrastructure on intertidal macrobenthic communities. Divers. Distrib. 22, 731–744. doi: 10.1111/ddi.12443
Dugan, J. E., Airoldi, L., Chapman, M. G., Walker, S. J., Schlacher, T., Wolanski, E., et al. (2011). “Estuarine and coastal structures: environmental effects, a focus on shore and nearshore structures,” in Treatise on Estuarine and Coastal Science, eds E. Wolanski and D. McLusky (London: Academic Press), 17–41. doi: 10.1016/B978-0-12-374711-2.00802-0
Dugan, J. E., Hubbard, D. M., Rodil, I. F., Revell, D. L., and Schroeter, S. (2008). Ecological effects of coastal armoring on sandy beaches. Mar. Ecol. 29, 160–170. doi: 10.1111/j.1439-0485.2008.00231.x
Englund, G., and Cooper, S. D. (2003). Scale effects and extrapolation in ecological experiments. Adv. Ecol. Res. 33, 161–213.
Evans, A. J., Firth, L. B., Hawkins, S. J., Hall, A. E., Ironside, J. E., Thompson, R. C., et al. (2019). From ocean sprawl to blue-green infrastructure–A UK perspective on an issue of global significance. Environ. Sci. Policy 91, 60–69. doi: 10.1016/S0065-2504(03)33011-9
Evans, A. J., Firth, L. B., Hawkins, S. J., Morris, E. S., Goudge, H., and Moore, P. J. (2016). Drill-cored rock pools: an effective method of ecological enhancement on artificial structures. Mar. Freshw. Res. 67, 123–130. doi: 10.1071/MF14244
Evans, A. J., Garrod, B., Firth, L. B., Hawkins, S. J., Morris-Webb, E. S., Goudge, H., et al. (2017). Stakeholder priorities for multi-functional coastal defence developments and steps to effective implementation. Mar. Policy 75, 143–155. doi: 10.1016/j.marpol.2016.10.006
Ferrario, F., Iveša, L., Jaklin, A., Perkol-Finkel, S., and Airoldi, L. (2016). The overlooked role of biotic factors in controlling the ecological performance of artificial marine habitats. J. Appl. Ecol. 53, 16–24. doi: 10.1111/1365-2664.12533
Firth, L. B., Airoldi, L., Bulleri, F., Challinor, S., Chee, S. Y., Evans, A. J., et al. (2020). Greening of grey infrastructure should not be used as a Trojan horse to facilitate coastal development. J. Appl. Ecol. 57, 1762–1768. doi: 10.1111/1365-2664.13683
Firth, L. B., Duff, L., Gribben, P. E., and Knights, A. M. (2021). Do positive interactions between marine invaders increase likelihood of invasion into natural and artificial habitats? Oikos 130, 453–463. doi: 10.1111/oik.07862
Firth, L. B., Knights, A. M., Bridger, D., Evans, A., Mieskowska, N., Moore, P. J., et al. (2016a). “Ocean sprawl: challenges and opportunities for biodiversity management in a changing world,” in Oceanography and Marine Biology: An Annual Review, eds A. C. Dale, I. P. Smith, R. N. Hughes, and D. J. Hughes (Boca Raton: CRC Press), 193–269.
Firth, L. B., White, F. J., Schofield, M., Hanley, M. E., Burrows, M. T., Thompson, R. C., et al. (2016b). Facing the future: the importance of substratum features for ecological engineering of artificial habitats in the rocky intertidal. Mar. Freshw. Res. 67, 131–143. doi: 10.1071/MF14163
Firth, L. B., Thompson, R. C., White, F. J., Schofield, M., Skov, M. W., Hoggart, S. P., et al. (2013). The importance of water-retaining features for biodiversity on artificial intertidal coastal defence structures. Divers. Distrib. 19, 1275–1283. doi: 10.1111/ddi.12079
Gittman, R. K., Fodrie, F. J., Popowich, A. M., Keller, D. A., Bruno, J. F., Currin, C. A., et al. (2015). Engineering away our natural defenses: an analysis of shoreline hardening in the US. Front. Ecol. Environ. 13, 301–307. doi: 10.1890/150065
Gittman, R. K., Scyphers, S. B., Smith, C. S., Neylan, I. P., and Grabowski, J. H. (2016). Ecological consequences of shoreline hardening: a meta-analysis. Bioscience 66, 763–773. doi: 10.1093/biosci/biw091
Goodsell, P. J., Chapman, M. G., and Underwood, A. J. (2007). Differences between biota in anthropogenically fragmented habitats and in naturally patchy habitats. Mar. Ecol. Prog. Ser. 351, 15–23. doi: 10.3354/meps07144
Gratwicke, B., and Speight, M. R. (2005). The relationship between fish species richness, abundance, and habitat complexity in a range of shallow tropical marine habitats. J. Fish Biol. 66, 650–667. doi: 10.1111/j.0022-1112.2005.00629.x
Hadfield, M., and Paul, V. (2001). “Natural chemical cues for settlement and metamorphosis of marine-invertebrate larvae,” in Marine Chemical Ecology, eds J. B. McClintock and B. J. Baker (Boca Raton: CRC Press).
Hall, A. E., Herbert, R. J., Britton, J. R., Boyd, I. M., and George, N. C. (2019). Shelving the coast with vertipools: retrofitting artificial rock pools on coastal structures as mitigation for coastal squeeze. Front. Mar. Sci. 6:456. doi: 10.3389/fmars.2019.00456
Heery, E. C., Bishop, M. J., Critchley, L. P., Bugnot, A. B., Airoldi, L., Mayer-Pinto, M., et al. (2017). Identifying the consequences of ocean sprawl for sedimentary habitats. J. Exp. Mar. Biol. Ecol. 492, 31–48. doi: 10.1016/j.jembe.2017.01.020
Hoggart, S., Hawkins, S. J., Bohn, K., Airoldi, L., van Belzen, J., Bichot, A., et al. (2015). “Ecological approaches to coastal risk mitigation,” in Coastal Risk Management in a Changing Climate, ed. B. Zanuttigh, (Oxford: Butterworth-Heinemann), 171–236.
Hughes, D J., Cook, E. J., and Sayer, M. D. (2005). Biofiltration and biofouling on artificial structures in Europe: the potential for mitigating organic impacts. Oceanogr. Mar. Biol. 43, 123–172.
Iveša, L., Chapman, M. G., Underwood, A. J., and Murphy, R. J. (2010). Differential patterns of distribution of limpets on intertidal seawalls: experimental investigation of the roles of recruitment, survival and competition. Mar. Ecol. Prog. Ser. 407, 55–69. doi: 10.3354/meps08539
Kavanagh, C. J., Schultz, M. P., Swain, G. W., Stein, J., Truby, K., and Wood, C. D. (2001). Variation in adhesion strength of Balanus eburneus, Crassostrea virginica and hydroides dianthus to fouling-release coatings. Biofouling 17, 155–167. doi: 10.1080/08927010109378474
Kovalenko, K. E., Thomaz, S. M., and Warfe, D. M. (2012). Habitat complexity: approaches and future directions. Hydrobiologia 685, 1–17.
Lenth, R. V. (2020). emmeans: Estimated Marginal Means, aka Least-Squares Means. R package version 1.5.3.
Lim, C. S., Leong, Y. L., and Tan, K. S. (2017). Managing the risk of non-indigenous species transfer in Singapore using a study of vessel movement. Mar. Pollut. Bull. 115, 332–344. doi: 10.1016/j.marpolbul.2016.12.009
Lim, S. C., de Voogd, N. J., and Tan, K. S. (2009). Fouling sponges (Porifera) on navigation buoys from Singapore waters. Raffles Bull. Zool. 22, 41–58.
Loke, L. H. L., Eliza, C. H., Samantha, L., Tjeerd, J. B., and Peter, A. T. (2019). Area-independent effects of water-retaining features on intertidal biodiversity on eco-engineered seawalls in the tropics. Front. Mar. Sci. 6:16. doi: 10.3389/fmars.2019.00016
Martinez Arbizu, P. (2020). pairwiseAdonis: Pairwise multilevel comparison using adonis. R package version 0.4.
McAbendroth, L., Ramsay, P. M., Foggo, A., Rundle, S. D., and Bilton, D. T. (2005). Does macrophyte fractal complexity drive invertebrate diversity, biomass and body size distributions? Oikos 111, 279–290. doi: 10.1111/j.0030-1299.2005.13804.x
McAfee, D., Bishop, M. J., Yu, T. N., and Williams, G. A. (2018). Structural traits dictate abiotic stress amelioration by intertidal oysters. Funct. Ecol. 32, 2666–2677. doi: 10.1111/1365-2435.13210
McAfee, D., Cole, V. J., and Bishop, M. J. (2016). Latitudinal gradients in ecosystem engineering by oysters vary across habitats. Ecology 97, 929–939. doi: 10.1890/15-0651.1
McManus, R. S., Archibald, N., Comber, S., Knights, A. M., Thompson, R. C., and Firth, L. B. (2018). Partial replacement of cement for waste aggregates in concrete coastal and marine infrastructure: a foundation for ecological enhancement? Ecol. Eng. 120, 655–667.
Miller, R. J., and Etter, R. J. (2008). Shading facilitates sessile invertebrate dominance in the rocky subtidal Gulf of Maine. Ecology 89, 452–462. doi: 10.1890/06-1099.1
Mineur, F., Le Roux, A., Stegenga, H., Verlaque, M., and Maggs, C. A. (2012). Four new exotic red seaweeds on European shores. Biol. Invasions 14, 1635–1641.
Morris, R. L., Chapman, M. G., Firth, L. B., and Coleman, R. A. (2017). Increasing habitat complexity on seawalls: investigating large-and small-scale effects on fish assemblages. Ecol. Evol. 7, 9567–9579. doi: 10.1002/ece3.3475
Moschella, P. S., Abbiati, M., Åberg, P., Airoldi, L., Anderson, J. M., Bacchiocchi, F., et al. (2005). Low-crested coastal defence structures as artificial habitats for marine life: using ecological criteria in design. Coast. Eng. 52, 1053–1071. doi: 10.1016/j.coastaleng.2005.09.014
Naylor, J. R., and McShane, P. E. (1997). Predation by polychaete worms on larval and post-settlement abalone Haliotis iris (Mollusca: Gastropoda). J. Exp. Mar. Biol. Ecol. 214, 283–290. doi: 10.1016/S0022-0981(97)00030-0
Naylor, L. A., Kippen, H., Coombes, M. A., Horton, B., MacArthur, M., and Jackson, N. (2017). Greening the Grey: a framework for integrated green grey infrastructure (IGGI). Glasgow: University of Glasgow.
Nordstrom, K. F. (2014). Living with shore protection structures: a review. Estuar. Coast. Shelf Sci. 150, 11–23. doi: 10.1016/j.ecss.2013.11.003
Oksanen, J., Blanchet, F. G., Kindt, R., Legendre, P., Minchin, P. R., O’hara, R. B., et al. (2014). Vegan: Community ecology package. R package version 2.2-0.
O’Shaughnessy, K. A., Hawkins, S. J., Evans, A. J., Hanley, M. E., Lunt, P., Thompson, R. C., et al. (2020). Design catalogue for eco-engineering of coastal artificial structures: a multifunctional approach for stakeholders and end-users. Urban Ecosyst. 23, 431–443. doi: 10.1007/s11252-019-00924-z
O’Shaughnessy, K. A., Perkol-Finkel, S., Strain, E. M. A., Bishop, M. J., Hawkins, S. J., Hanley, M. E., et al. (2021). Spatially variable effects of artificially created physical complexity on subtidal benthos. Front. Ecol. Evol. 9:6904137. doi: 10.3389/fevo.2021.690413
Perkins, M. J., Ng, T. P., Dudgeon, D., Bonebrake, T. C., and Leung, K. M. (2015). Conserving intertidal habitats: what is the potential of ecological engineering to mitigate impacts of coastal structures? Estuar. Coast. Shelf Sci. 167, 504–515. doi: 10.1016/j.ecss.2015.10.033
Porri, F., Jordaan, T., and McQuaid, C. D. (2008). Does cannibalism of larvae by adults affect settlement and connectivity of mussel populations? Estuar. Coast. Shelf Sci. 79, 687–693. doi: 10.1016/j.ecss.2008.06.010
Röding, P.F. (1798). “Museum boltenianum sive catalogus cimeliorum e tribus regnis naturæ quæ olim collegerat Joa,” in Annos Proto Physicus Hamburgensis: Pars Secunda Continens Conchylia Sive Testacea Univalvia, Bivalvia & Multivalvia, ed. M. D. Fried Bolten (Hamburg: Trapp), viii, 199.
Russell, G., Hawkins, S. J., Evans, L. C., Jones, H. D., and Holmes, G. D. (1983). Restoration of a disused dock basin as a habitat for marine benthos and fish. J. Appl. Ecol. 20, 43–58. doi: 10.2307/2403375
Satheesh, S., and Wesley, S. G. (2010). Influence of substratum colour on the recruitment of macrofouling communities. J. Mar. Biol. Assoc. U. K. 90, 941–946. doi: 10.1017/S0025315410000032
Schotanus, J., Walles, B., Capelle, J. J., Van Belzen, J., Van De Koppel, J., and Bouma, T. J. (2020). Promoting self-facilitating feedback processes in coastal ecosystem engineers to increase restoration success: testing engineering measures. J. Appl. Ecol. 57, 1958–1968. doi: 10.1111/1365-2664.13709
Shin, L. Y., and Abllah, N. F. N. (2015). Sewerage catchment study for clan jetties, Penang. J. Teknol. 75, 101–105. doi: 10.11113/jt.v75.5078
Strain, E., Morris, R., and Bishop, M. (2017). Sydney Harbour: Enhancing seawall sustainability. Australia: NSW Department of Primary Industries.
Strain, E. M., Olabarria, C., Mayer-Pinto, M., Cumbo, V., Morris, R. L., Bugnot, A. B., et al. (2018). Eco-engineering urban infrastructure for marine and coastal biodiversity: which interventions have the greatest ecological benefit?. J. Appl. Ecol. 55, 426–441. doi: 10.1111/1365-2664.12961
Strain, E. M., Steinberg, P. D., Vozzo, M., Johnston, E. L., Abbiati, M., Aguilera, M. A., et al. (2021). A global analysis of complexity–biodiversity relationships on marine artificial structures. Glob. Ecol. Biogeogr. 30, 140–153. doi: 10.1111/geb.13202
Strain, E. M. A., Cumbo, V. R., Morris, R. L., Steinberg, P. D., and Bishop, M. J. (2020). Interacting effects of habitat structure and seeding with oysters on the intertidal biodiversity of seawalls. PLoS One 15:e0230807. doi: 10.1371/journal.pone.0230807
Taira, D., Heery, E. C., Loke, L. H., Teo, A., Bauman, A. G., and Todd, P. A. (2020). Ecological engineering across organismal scales: trophic-mediated positive effects of microhabitat enhancement on fishes. Mar. Ecol. Prog. Ser. 656, 181–192. doi: 10.3354/meps13462
Taylor, P. D., and Tan, S. H. A. (2015). Cheilostome Bryozoa from Penang and Langkawi, Malaysia. Eur. J. Taxon. 149, 1–34. doi: 10.5852/ejt.2015.149
Todd, P. A., Heery, E. C., Loke, L. H., Thurstan, R. H., Kotze, D. J., and Swan, C. (2019). Towards an urban marine ecology: characterizing the drivers, patterns and processes of marine ecosystems in coastal cities. Oikos 128, 1215–1242. doi: 10.1111/oik.05946
Trygonis, V., and Sini, M. (2012). photoQuad: a dedicated seabed image processing software, and a comparative error analysis of four photoquadrat methods. J. Exp. Mar. Biol. Ecol. 424, 99–108. doi: 10.1016/j.jembe.2012.04.018
Ushiama, S., Mayer-Pinto, M., Bugnot, A. B., Johnston, E. L., and Dafforn, K. A. (2019). Eco-engineering increases habitat availability and utilisation of seawalls by fish. Ecol. Eng. 138, 403–411. doi: 10.1016/j.ecoleng.2019.07.022
Vieira, E. A., Flores, A. A., and Dias, G. M. (2018). Persistence and space preemption explain species-specific founder effects on the organization of marine sessile communities. Ecol. Evol. 8, 3430–3442. doi: 10.1002/ece3.3853
Vozzo, M. L., Mayer-Pinto, M., Bishop, M. J., Cumbo, V. R., Bugnot, A. B., Dafforn, K. A., et al. (2021). Making seawalls multifunctional: the positive effects of seeded bivalves and habitat structure on species diversity and filtration rates. Mar. Environ. Res. 165:105243. doi: 10.1016/j.marenvres.2020.105243
Waltham, N. J., and Dafforn, K. A. (2018). Ecological engineering in the coastal seascape. Ecol. Eng. 120, 554–559. doi: 10.1016/j.ecoleng.2018.07.028
Waltham, N. J., and Sheaves, M. (2015). Expanding coastal urban and industrial seascape in the Great Barrier Reef World Heritage Area: critical need for coordinated planning and policy. Mar. Policy 57, 78–84. doi: 10.1016/j.marpol.2015.03.030
Wells, F. E., Tan, K. S., Todd, P. A., Jaafar, Z., and Yeo, D. C. (2019). A low number of introduced marine species in the tropics: a case study from Singapore. Manag. Biol. Invasions 10, 23–45. doi: 10.3391/mbi.2019.10.1.03
Yeager, M. E., and Hovel, K. A. (2017). Structural complexity and fish body size interactively affect habitat optimality. Oecologia 185, 257–267. doi: 10.1007/s00442-017-3932-2
Keywords: nature-based solutions, ecological engineering, complexity, greening-of-grey infrastructure, blue-green infrastructure, transplant
Citation: Chee SY, Yee JC, Cheah CB, Evans AJ, Firth LB, Hawkins SJ and Strain EMA (2021) Habitat Complexity Affects the Structure but Not the Diversity of Sessile Communities on Tropical Coastal Infrastructure. Front. Ecol. Evol. 9:673227. doi: 10.3389/fevo.2021.673227
Received: 27 February 2021; Accepted: 14 September 2021;
Published: 04 October 2021.
Edited by:
Tao Li, Sichuan University, ChinaReviewed by:
Gustavo Muniz Dias, Federal University of ABC, BrazilKoh Siang Tan, National University of Singapore, Singapore
Copyright © 2021 Chee, Yee, Cheah, Evans, Firth, Hawkins and Strain. This is an open-access article distributed under the terms of the Creative Commons Attribution License (CC BY). The use, distribution or reproduction in other forums is permitted, provided the original author(s) and the copyright owner(s) are credited and that the original publication in this journal is cited, in accordance with accepted academic practice. No use, distribution or reproduction is permitted which does not comply with these terms.
*Correspondence: Su Yin Chee, suyinchee@usm.my